Abstract
A whole-body physiologically-based model was developed to describe the pharmacokinetics of the ansamycin benzoquinone antibiotic 17-(allylamino)-17-demethoxygeldanamycin (17AAG) and its active metabolite 17-(amino)-17-demethoxygeldanamycin (17AG) in blood, normal organs (lung, brain, heart, spleen, liver, kidney, skeletal muscle) and implanted human tumor xenograft in nude mice. The distribution of 17AAG in all organs was described by diffusion-limited exchange models, while that of 17AG was described by perfusion-limited models. The intrinsic clearances of 17AAG and 17AG in the liver were uniquely identified using local models and were estimated to be 4.93 ml/hr and 3.34 ml/hr. It was also estimated that the formation of 17AG in liver accounted for 40% of the 17AAG intrinsic clearance. The model for the distribution of both 17AAG and 17AG in the human breast cancer tumor xenograft included vascular, interstitial and intracellular compartments, which yielded the predicted cellular concentrations of 17AAG and 17AG two to three times higher than the corresponding whole tissue measurements at steady state. Estimates of the vascular-interstitial permeability surface-area product were similar for 17AAG and 17AG (0.23 ml/hr and 0.26 ml/hr). However, the interstitial to cellular transport rate of 17AG was three-fold greater than that of 17AAG, which resulted in the preferential uptake of 17AG over 17AAG in tumor. Indirect response models were developed to describe the combined action of 17AAG and 17AG on the onco-proteins Raf-1 and p185erbB2 in tumor. The half-life of endogenous protein turnover was estimated to be 22.6 hr for Raf-1 and 8.6 hr for p185erbB2, and both were comparable to corresponding values measured in vitro. A model for the molecular chaperon heat shock proteins HSP70 and HSP90 was developed based on the molecular mechanism of heat shock auto-regulation and the action of 17AAG and 17AG on these proteins. The model provided in vivo estimates of endogenous HSP70 and HSP90 turnover. In modeling pharmacokinetics and pharmacodynamics, Bayesian inference was employed to estimate the kinetic, physiological and molecular parameters when prior information was available.
Similar content being viewed by others
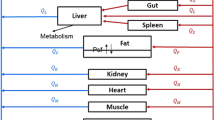
References
C. Erlichman, J. L. Grem, H. I. Scher, D. L. Trump, R. Ramanathan, M. J. Egorin, B. Blaylock, and P. Ivy. Phase I clinical trials of 17-allylamino-17-deoxygeldanamycin (17-AAG) (NSC#330507) sponsored by the national cancer institute, USA(NCI). 1st International Conference on the HSP90 Chaperone Machine. (2002)
P. N. Munster, M. Srethapakdi, M. M. Moasser, and N. Rosen. Inhibition of heat shock protein 90 function by ansamycins causes the morphological and functional differentiation of breast cancer cells. Cancer Res. 61:2945–2952 (2001).
R. C. Schnur, M. L. Corman, R. J. Gallaschun, B. A. Cooper, M. F. Dee, J. L. Doty, M. L. Muzzi, J. D. Moyer, C. I. DiOrio, E. G. Barbacci, P. E. Miller, A. T. O'Brien, M. J. Morin, B. A. Foster, V. A. Pollack, D. M. Savage, D. E. Sloan, L. R. Pustilnik, and M. P. Moyer. Inhibition of the oncogene product p185erbB-2 in vitro and in vivo by geldanamycin and dihydrogeldanamycin derivatives. J. Med. Chem. 38:3806–3812 (1995).
R. C. Schnur, M. L. Corman, R. J. Gallaschun, B. A. Cooper, M. F. Dee, J. L. Doty, M. L. Muzzi, C. I. DiOrio, E. G. Barbacci, P. E. Miller, V. A. Pollack, D. M. Savage, D. E. Sloan, L. R. Pusitilnik, J. D. Mayer, and M. P. Moyer. erbB-2 oncogene inhibition by geldanamycin derivatives: synthesis, mechanism of action, and structure-activity relationships. J. Med. Chem. 38:3813–3820 (1995).
T. W. Schulte and L. M. Neckers. The benzoquinone ansamycin 17-allylamino-17-demethoxygeldanamycin binds to HSP90 and shares important biologic activities with geldanamycin. Cancer Chemother. Pharmacol. 42:273–279 (1998).
J. Page, J. Heath, R. Fulton, E. Yalkowsky, E. Tabibi, J. Tomzszewski, A. Smith, and L. Rodman. Comparison of geldanamycin (NSC-122750) and 17-allylaminogeldanamycin (NSC-330507D) toxicity in rats. Proc. Am. Assoc. Cancer Res. 38:308(1997).
M. J. Egorin, D. M. Rosen, J. H. Wolff, P. S. Callery, S. M. Musser, and J. L. Eiseman. Metabolism of 17-(allylamino)-17-demethoxygeldanamycin (NSC 330507) by murine and human hepatic preparations. Cancer Res. 58:2385–2396 (1998).
M. J. Egorin, E. G. Zuhowski, D. M. Rosen, D. L. Sentz, J. M. Covey, and J. L. Eiseman. Plasma pharmacokinetics and tissue distribution of 17-(allylamino)-17-demethoxy-geldanamycin (NSC 330507) in CD2F1 mice1. Cancer Chemother. Pharmacol. 47:291–302 (2001).
W. G. An, R. C. Schnur, L. M. Neckers, and M. V. Blagosklonny. Depletion of p185erbB2, Raf-1 and mutant p53 proteins by geldanamycin derivatives correlates with antiproliferative activity. Cancer Chemother. Pharmacol. 40:60–64 (1997).
L. M. Neckers, T. W. Schulte, and E. Mimnaugh. Geldanamycin as a potential anti-cancer agent: its molecular target and biochemical activity. Inûest. New Drugs 17:361–373 (1999).
C. Chavany, E. Mimnaugh, P. Miller, R. Bitton, P. Nguyen, J. Trepel, L. Whitesell, R. Schnur, J. Moyer, and L. Neckers. p185erbB2 binds to GRP94 in vivo. Dissociation of the p185erbB2/GRP94 heterocomplex by benzoquinone ansamycins precedes depletion of p185erbB2}. J. Biol. Chem. 271:4974–4977 (1996).
E. G. Mimnaugh, C. Chavany, and L. Neckers. Polyubiquitination and proteasomal degradation of the p185c-erbB-2 receptor protein-tyrosine kinase induced by geldanamycin. J. Biol. Chem. 271:22796–22801 (1996).
C. Schneider, L. Sepp-Lorenzino, E. Nimmesgern, O. Ouerfelli, S. Danishefsky, N. Rosen, and F. U. Hartl. Pharmacologic shifting of a balance between protein refolding and degra-dation mediated by Hsp90. Proc. Natl. Acad. Sci. 93:14536–14541 (1996).
T. W. Schulte, M. V. Blagosklonny, C. Ingui, and L. Neckers. Disruption of the Raf-1-Hsp90 molecular complex results in destabilization of Raf-1 and loss of Raf-1-Ras association. J. Biol. Chem. 270:24585–24588 (1995).
C. E. Stebbins, A. A. Russo, C. Schneider, N. Rosen, F. U. Hartl, and N. P. Pavletich. Crystal structure of an Hsp90-geldanamycin complex: targeting of a protein chaperone by an antitumor agent. Cell 89:239–250 (1997).
A. Sharma and W. J. Jusko. Characterization of four basic models of indirect pharmacodynamic responses. J. Pharmacokinet. Biopharm. 24:611–635 (1996).
R. I. Morimoto. Cells in stress: transcriptional activation of heat shock genes. Science 259:1409–1410 (1993).
C. Jolly and R. I. Morimoto. Role of the heat shock response and molecular chaperones in oncogenesis and cell death. J. Natl. Cancer Inst. 92:1564–1572 (2000).
Y. Shi, D. D. Mosser, and R. I. Morimoto. Molecular chaperones as HSF1-specific tran-scriptional repressors. Genes Dev. 12:654–666 (1998).
R. I. Morimoto. Regulation of the heat shock transcriptional response: cross talk between a family of heat shock factors, molecular chaperones, and negative regulators. Genes Dev. 12:3788–3796 (1998).
D. E. Mager and W. J. Jusko. Pharmacodynamic modeling of time-dependent transduction systems. Clin. Pharmacol. Ther. 70:210–216 (2001).
D. Z. D'Argenio and A. Schumitzky. ADAPT II User's Guide: Pharmacokinetic/Pharmacodynamic Systems Analysis Software. Biomedical Simulations Resource, Los Angeles, 1997.
P. Wang, Z. F. Ba, J. Burkhardt, and I. H. Chaudry. Trauma-hemorrhage and resusci-tation in the mouse: effects on cardiac output and organ blood flow. Am. J. Physiol. 264:H1166–1173 (1993).
R. W. Barbee, B. D. Perry, R. N. Re, and J. P. Murgo. Microsphere and dilution tech-niques for the determination of blood flows and volumes in conscious mice. Am. J. Physiol. 263:R728–733 (1992).
R. P. Brown, M. D. Delp, S. L. Lindstedt, L. R. Rhomberg, and R. P. Beliles. Physiological parameter values for physiologically based pharmacokinetic models. Toxicol. Ind. Health 13:407–484 (1997).
M. J. Mantyla. Regional blood flow in human tumors. Cancer Res. 39:2304–2306 (1979).
R. K. Jain. Determinants of tumor blood flow: a review. Cancer Res. 48:2641–2658 (1988).
L. T. Baxter, H. Zhu, D. G. Mackensen, and R. K. Jain. Physiologically based pharmaco-kinetic model for specific and nonspecific monoclonal antibodies and fragments in normal tissues and human tumor xenografts in nude mice. Cancer Res. 54:1517–1528 (1994).
R. K. Jain. Transport of molecules in the tumor interstitium: a review. Cancer Res. 47:3039–3051 (1987).
W. Xu, E. Mimnaugh, M. F. Rosser, C. Nicchitta, M. Marcu, Y. Yarden, and L. Neckers. Sensitivity of mature P185erbB2 to geldanamycin is conferred by its kinase domain and is mediated by the chaperone protein Hsp90. J. Biol. Chem. 276:3702–3708 (2001).
P. Miller, C. DiOrio, M. Moyer, R. C. Schnur, A. Bruskin, W. Cullen, and J. D. Moyer. Depletion of the erbB-2 gene product p185 by benzoquinoid ansamycins. Cancer Res. 54:2724–2730 (1994).
G. E. Blakey, I. A. Nestorov, P. A. Arundel, L. J. Aarons, and M Rowland. Qualitative Structure-Pharmacokinetics Relationships: I. Development of a Whole-Body Physiologically Based Model to Characterize Changes in Pharmacokinetics Across a Homologous Series of Barbuturates in the Rat. J. Pharmacokin. Biopharm. 25:277–213 (1997).
A. Gelman, F. Y. Bois, and J. Jiang. Physiological pharmacokinetic analysis using popu-lation modeling and informative prior distributions. J. Am. Stat. Assoc. 91:1400–1412 (1996).
F. Jonsson and G. Johanson. Physiologically based modeling of the inhalation kinetics of styrene in humans using a Bayesian population approach. Toxicol. Appl. Pharmacol. 179:35–49 (2002).
Author information
Authors and Affiliations
Corresponding author
Rights and permissions
About this article
Cite this article
Xu, L., Eiseman, J.L., Egorin, M.J. et al. Physiologically-Based Pharmacokinetics and Molecular Pharmacodynamics of 17-(allylamino)-17-demethoxygeldanamycin and Its Active Metabolite in Tumor-Bearing Mice. J Pharmacokinet Pharmacodyn 30, 185–219 (2003). https://doi.org/10.1023/A:1025542026488
Issue Date:
DOI: https://doi.org/10.1023/A:1025542026488