Signatures of Altered Gene Expression in Dorsal Root Ganglia of a Fabry Disease Mouse Model
- Division of Physiology, Department of Physiology and Medical Physics, Medical University of Innsbruck, Innsbruck, Austria
Fabry disease is an X-linked lysosomal storage disorder with involvement of the nervous system. Accumulation of glycosphingolipids within peripheral nerves and/or dorsal root ganglia results in pain due to small-fiber neuropathy, which affects the majority of patients already in early childhood. The α-galactosidase A deficient mouse proved to be an adequate model for Fabry disease, as it shares many symptoms including altered temperature sensitivity and pain perception. To characterize the signatures of gene expression that might underlie Fabry disease-associated sensory deficits and pain, we performed one-color based hybridization microarray expression profiling of DRG explants from adult α-galactosidase A deficient mice and age-matched wildtype controls. Protein-protein interaction (PPI) and pathway analyses were performed for differentially regulated mRNAs. We found 812 differentially expressed genes between adult α-galactosidase A deficient mice and age-matched wildtype controls, 506 of them being upregulated, and 306 being downregulated. Among the enriched pathways and processes, the disease-specific pathways “lysosome” and “ceramide metabolic process” were identified, enhancing reliability of the current analysis. Novel pathways that we identified include “G-protein coupled receptor signaling” and “retrograde transport” for the upregulated genes. From the analysis of downregulated genes, immune-related pathways, autoimmune, and infection pathways emerged. The current analysis is the first to present a differential gene expression profile of DRGs from α-galactosidase A deficient mice, thereby providing knowledge on possible mechanisms underlying neuropathic pain related symptoms in Fabry patients. Therefore, the presented data provide new insights into the development of the pain phenotype and might lead to new treatment strategies.
Introduction
Fabry disease (FD) is an X-linked lysosomal storage disorder with estimated incidence rates of 1:37,000 for the classical Fabry phenotype and 1:3,100 for a late-onset disease variant (Spada et al., 2006; Mechtler et al., 2012). It can be caused by more than 500 different mutations of the lysosomal α-galactosidase A (α-Gal A) gene (Gal et al., 2006; Saito et al., 2011). Those mutations lead to deficient activity, reduction or depletion of α-Gal A, followed by impaired degradation of glycosphingolipids and subsequent accumulation of globotriaosylceramide (Gb3) in a variety of tissues, including vascular endothelial cells and neurons (Desnick et al., 2001; Bangari et al., 2015). In general, males are more affected by the α-Gal A mutations, but also heterozygote females have a significant risk for major organ involvement due to random X-inactivation causing variable expression of α-Gal A and decreased quality of life (Wilcox et al., 2008). One of the earliest symptoms of FD is pain due to small-fiber neuropathy, which affects the majority of patients already in early childhood. It can manifest as episodic crises with pain attacks originating in the extremities that can last for several days or even weeks, or chronic pain characterized by burning and tingling paraesthesia (Germain, 2010; Ginsberg, 2013). The origin of this pain phenotype presumably lies in accumulation of glycolipids within peripheral nerves and/or dorsal root ganglion (DRG) somata that might lead to degeneration of small sensory fibers (Kocen and Thomas, 1970; Ohnishi and Dyck, 1974; Bangari et al., 2015; Godel et al., 2017).
Investigating FD specific pathogenesis in Fabry patients is difficult and limited to molecular analyses of tissue biopsies and clinical neurophysiology techniques. It has been found that both motor and sensory conduction velocities are decreased, whereas vibratory, cold, and heat thresholds are elevated in Fabry patients (Sheth and Swick, 1980; Dutsch et al., 2002; Uceyler et al., 2013; Namer et al., 2017). In addition, the proportion of mechano-responsive C-fibers is reduced in patients compared to healthy controls (Namer et al., 2017). To investigate the molecular and physiological mechanisms underlying the pathology of FD, α-galactosidase A deficient mice [α-Gal A(−/0)] were generated which share many symptoms with Fabry patients, including altered temperature sensitivity and pain perception (Ohshima et al., 1997; Lakoma et al., 2014; Uceyler et al., 2016; Namer et al., 2017).
Although FD constitutes a monogenic disease with loss of function mutations of the α-Gal A gene causing the disease, other genes and/or gene products might be indirectly regulated during disease progression and could play important roles in the manifestation of disease-specific pathologies and symptoms, like the development of small-fiber neuropathy. In the current study we therefore performed mRNA microarray expression profiling of DRG samples from α-Gal A(−/0) mice aged > 20 weeks when the disease is fully developed to investigate the mRNA signatures associated with FD peripheral nerve neuropathy.
Methods
Animals
Male α-galactosidase A(−/0) (α-Gal A(−/0); background C57BL/6; provided by Dr. A. Kulkarni, National Institute of Health, NIDCR, Bethesda, USA) (Ohshima et al., 1997) and wildtype C57BL/6J mice aged 20-24 weeks were inbred and housed under specific pathogen-free (SPF) conditions. For microarray expression profiling mice from the separate inbred colonies were used, whereas for RT-qPCR validation, α-Gal A(−/0) mice backcrossed with wildtype C57BL/6J mice and wildtype C57BL/6J mice were used to control for inbred colony effects. Animals were maintained at constant room temperature of 24°C on a 12 h light/dark cycle with lights on from 07:00 to 19:00 and had ad libitum access to autoclaved pelleted food and water. All animals were treated in accordance with the Ethics Guidelines of Animal Care (Medical University of Innsbruck), as well as the European Communities Council Directive of 22 September 2010 on the protection of animals used for scientific purposes (2010/63/EU), and approved by the Austrian National Animal Experiment Ethics Committee of the Austrian Bundesministerium für Wissenschaft und Forschung (permit number BMWF-66.011/0054-WF/V/3b/2015).
Tissue Collection
For microarray expression profiling eight adult mice (aged between 20 and 24 weeks) per group, whereas for RT-qPCR validation six adult mice (aged between 20 and 24 weeks) per group, were deeply anesthetized with isoflurane and euthanized by decapitation. Spinal cords were removed, lumbar DRGs L3-L5 (containing the cell bodies of primary afferents that project into the hind paw) harvested and flash-frozen in liquid nitrogen. Samples were kept at −80°C until further processing. For microarray expression profiling, DRGs from two mice were pooled for the final tissue sample.
Microarray Expression Profiling
Genome-wide expression profiling was carried out by IMGM Laboratories (Munich, Germany) using Agilent SurePrint G3 Mouse GE 8 × 60K Microarrays in combination with a one-color based hybridization protocol. Microarray signals were detected using the Agilent DNA Microarray Scanner.
Total RNA including small RNAs was isolated using the miRNeasy Mini Kit (Qiagen) according to the manufacturer's instructions and eluted in 40 μl RNase-free water. RNA concentration and purity was determined on a NanoDrop ND-1000 spectral photometer (Peqlab). Samples were analyzed using the RNA 6000 Nano LabChip Kit (Agilent Technologies) on a 2100 Bioanalyzer (Agilent Technologies). For mRNA analysis, total RNA samples were spiked with in vitro synthesized polyadenylated transcripts (One-Color RNA Spike-In Mix, Agilent Technologies), reverse transcribed into cDNA and then converted into Cyanine-3 labeled complementary RNA (Low Input Quick-Amp Labeling Kit One-Color, Agilent Technologies) according to the manufacturer's instructions. cRNA concentration, RNA absorbance ratio, and Cyanine-3 dye concentration were recorded using a NanoDrop ND-1000 UV-VIS spectral photometer, and quality of labeled cRNA was analyzed using the RNA 6000 Nano LabChip Kit (Agilent Technologies) on a 2100 Bioanalyzer (Agilent Technologies). Following cRNA clean-up and quantification, Cyanine-3-labeled cRNA samples were fragmented and prepared for one-color-based hybridization (Gene Expression Hybridization Kit, Agilent Technologies) and hybridized at 65°C for 17 h on Agilent SurePrint G3 Mouse GE 8 × 60K Microarrays. After hybridization, microarrays were washed with increasing stringency using Triton X-102 supplemented Gene Expression Wash Buffers (Agilent Technologies) followed by drying with acetonitrile (Sigma). Fluorescence signals were detected on an Agilent DNA Microarray Scanner and extracted using feature extraction software (Agilent Technologies). The data discussed in this publication have been deposited in NCBI's Gene Expression Omnibus (Edgar et al., 2002) and are accessible through GEO Series accession number GSE104625 (https://www.ncbi.nlm.nih.gov/geo/query/acc.cgi?acc=GSE104625).
Bioinformatics Analyses
GeneSpring GX 13.0 analysis software (Agilent Technologies) was used to normalize and analyze the microarray raw data. Data were normalized using non-parametric quantile normalization. Groups were compared using Welch's approximate t-test (unpaired unequal variances) and p-values corrected for multiple testing using the algorithm of Benjamini and Hochberg (Benjamini and Hochberg, 1995), controlling for false discovery rate (FDR). Differential expression between the two groups was determined by calculating fold changes of the averaged normalized expression values. Significantly regulated mRNAs were identified by applying filters on fold changes (absolute fold change ≥ 1.2 or ≥2) and p-values (p ≤ 0.01). Chip array data were further processed by R statistics statistical software package (R Development Core Team, 2008) and Volcano plots prepared using R statistics ggplot function. Only genes with uncompromised hybridization values in all individual samples were used for the current analysis.
Protein-Protein Interaction Analysis
Protein-protein interactions (PPIs) were investigated for the significantly regulated mRNAs using the STRING Database v. 10.5 (http://www.string-db.org) (Szklarczyk et al., 2017), which includes direct and indirect protein associations collected from different databases. Interaction networks were prepared using medium confidence scores (0.40) and clustered using MCL clustering algorithm (inflation parameter: 3). Disconnected nodes were hidden from the network.
Functional Enrichment and Pathway Analysis
Functional enrichment and pathway analyses were also performed using the STRING Database v. 10.5 (http://www.string-db.org). Classification systems tested were Gene Ontology and KEGG functional annotation spaces, employing Fisher's exact test followed by a correction for multiple testing (FDR). Only enriched pathways with FDR corrected p < 0.05 are reported.
RT-qPCR Validation of Regulated Genes
Reverse transcription quantitative polymerase chain reaction (RT-qPCR) validation of regulated genes was performed using TaqMan Gene Expression Assays (Thermo Fisher Scientific) in an Applied Biosystems 7500 Fast Real-Time PCR System (Thermo Fisher Scientific).
Total RNA was extracted using peqGOLD TriFast reagent (Peqlab) according to the manufacturer's instructions. The quality and quantity of RNA was evaluated using NanoDrop 2000 (Thermo Scientific). Reverse transcription of mRNA was performed as previously described (Langeslag et al., 2014). Genes of interest were analyzed by RT-qPCR using the following TaqMan Gene Expression Assays (Thermo Fisher Scientific): Mm00557794_m1 (Amz1), Mm00476032_m1 (Atf3), Mm01299527_m1 (Dnah8), Mm01311685_m1 (Dnase1l3), Mm00469610_m1 (Ecel1), Mm00521881_m1 (Meig1), Mm00505317_m1 (Ncapg2), Mm00443523_m1 (Opn4), Mm01279059_m1 (Rnf39), Mm00509406_m1 (Samd8), Mm00555659_m1 (Dock4), Mm03646971_gH (Gm1987), Mm02391771_g1 (Hdac1), Mm00440480_m1 (Nnat), Mm00452229_m1 (Pmepa1), Mm01188211_m1 (S100pbp), Mm00521530_m1 (Slc51a), Mm00628467_m1 (Syt15), Mm00503605_m1 (Tmem25), Mm00836474_m1 (Zfp932), Mm00446968_m1 (Hprt), Mm01352363_m1 (Sdha), and Mm00441941_m1 (Tfrc). Experimental procedures were performed according to the TaqMan Gene Expression Assays protocol. The reactions were loaded on MicroAmp Fast Optical 96-well reaction plates (Thermo Fisher Scientific) and placed in the Applied Biosystems 7500 Fast Real-Time PCR System (Thermo Fisher Scientific). The PCR cycle protocol used was: 10 min at 95°C, 40 two-step cycles of 15 s at 95°C and 1 min at 60°C. Each sample was run in duplicates alongside non-template controls. Threshold was set manually at 0.1 and threshold cycle (CT) was used as a measure of initial RNA input. Relative fold changes in gene expression were calculated using the 2−ΔΔCT method. All fold changes were expressed relative to the respective expression in wildtype mice and analyzed using Welch's t-test. Three genes (i.e., Hprt, Sdha and Tfrc) were used as reference genes. All three reference genes were found to be stably expressed in both groups of animals, as indicated by geNorm, Normfinder, and Bestkeeper software packages.
Results
mRNA Expression Profile of Fabry Mouse Dorsal Root Ganglia
Using microarray expression profiling we found that in total 812 genes from the overall 21,736 detected mRNAs were significantly different between DRG samples from wildtype and α-Gal A(−/0) mice (criteria p ≤ 0.01, absolute fold change ≥ 1.2) (Figure 1). Of those, 506 genes were significantly upregulated and 306 genes were significantly downregulated as compared to wildtype controls. More stringent filtering (criteria p ≤ 0.01, absolute fold change ≥ 2) of those significantly regulated genes revealed an assessable number of 78 genes in total (Figure 2). Using these criteria 41 genes were significantly upregulated, of which 29 showed FDR corrected p ≤ 0.1 (Table 1). Furthermore, 31 genes remained significantly downregulated, of which 27 showed FDR corrected p ≤ 0.1 (Table 2). PPI analysis (STRING Database) neither revealed clusters of interacting proteins nor enriched pathways, due to the small number of input genes. Thus, for in depth PPI analysis all significantly regulated genes (less stringent filtering, criteria p ≤ 0.01, absolute fold change ≥ 1.2) were used.
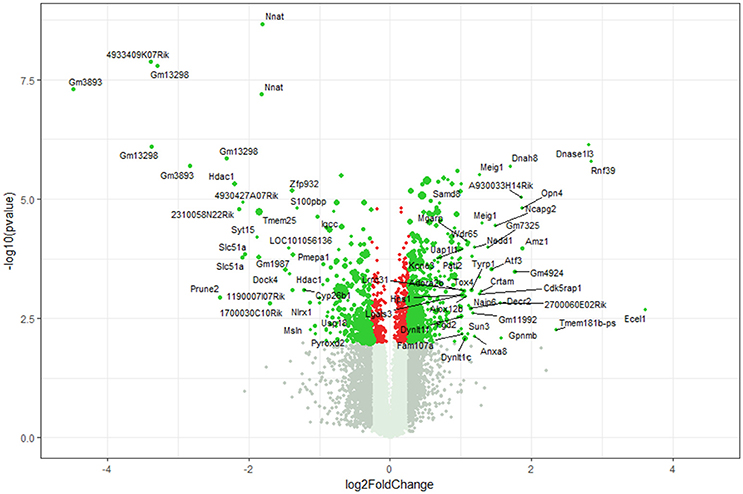
Figure 1. Volcano plot microarray data. Color green, p ≤ 0.01, fold change ≥ 1.2; labels, p ≤ 0.01, fold change ≥ 2.0; dot size represents relative expression values of wildtype mice.
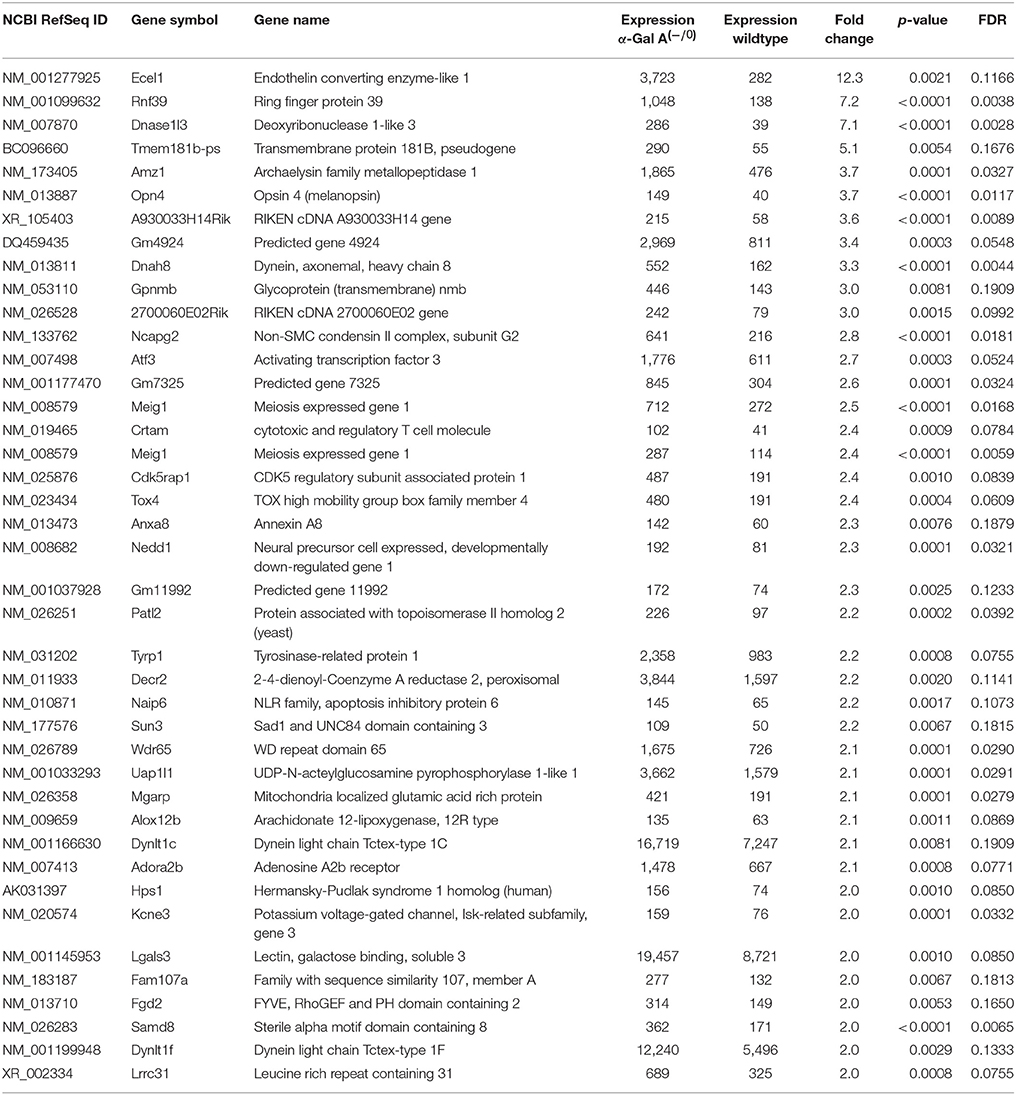
Table 1. Raw expression values, fold changes and statistical analysis for significantly upregulated genes.
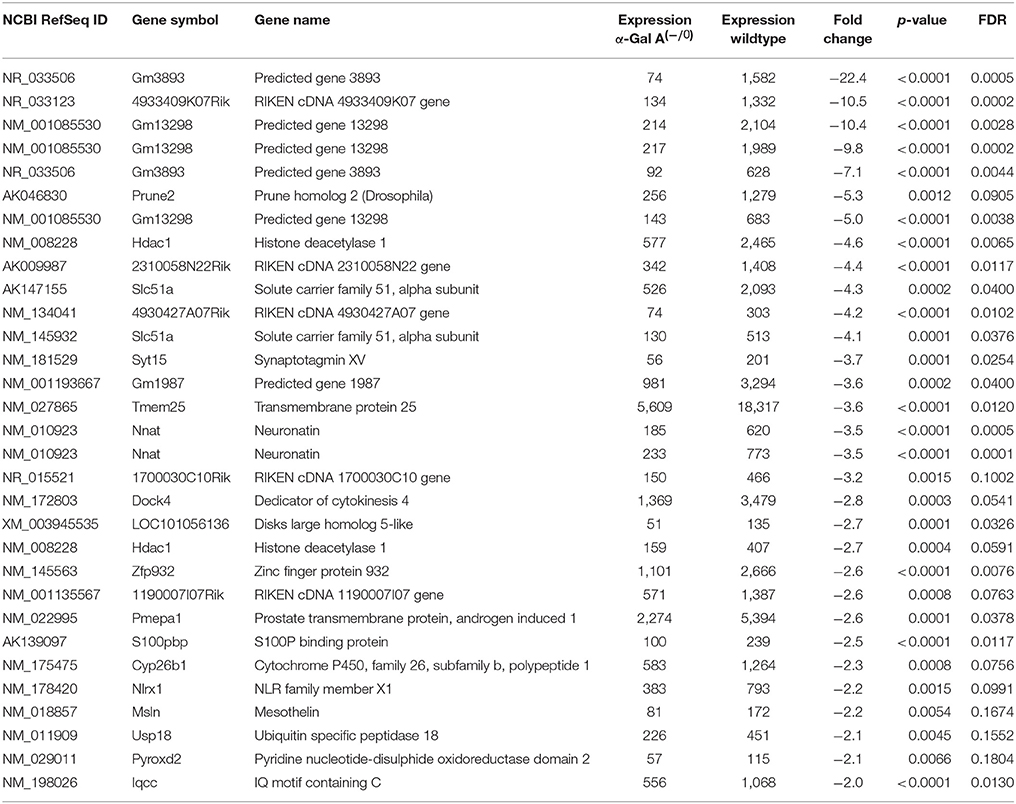
Table 2. Raw expression values, fold changes, and statistical analysis for significantly downregulated genes.
Enriched Pathways and Protein-Protein interactions for Upregulated mRNAs
Enrichment analysis of the 506 significantly upregulated genes revealed that a number of KEGG pathways and Gene Ontology processes were enriched, including the KEGG pathway “Lysosome” (KEGG:04142) and the biological process “Ceramide metabolic process” (GO:0006672), both known to constitute major hallmarks of FD pathogenesis (Table 3).
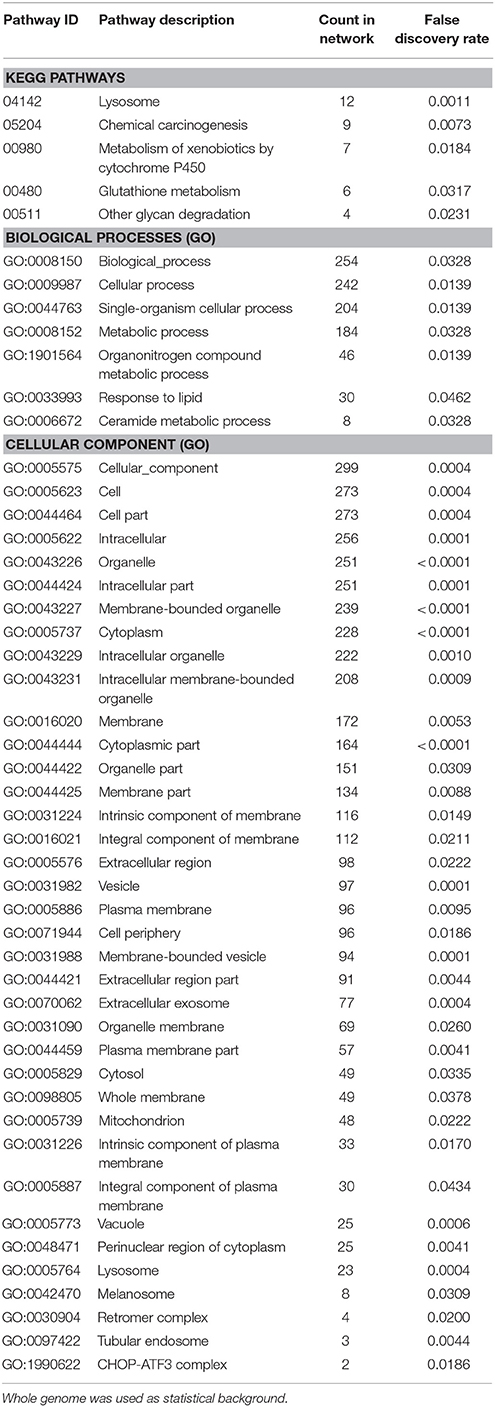
Table 3. Enrichment-analysis for upregulated mRNAs in α-Gal A(−/0) vs. wildtype mice using gene ontology and KEGG pathway annotations.
Protein-protein interaction (PPI) analysis of significantly upregulated mRNAs revealed a significant PPI enrichment (p < 0.0001; Figure 3). The number of actually observed edges (n = 328) exceeded the expected number of edges (n = 231) by 42%. Furthermore, three clusters of at least five interconnected proteins became apparent. Enrichment analysis of those clusters showed that those genes were involved in different pathways (Table 4), the red cluster was related to “G-protein coupled receptor signaling” (e.g., GO:0007186), the pink cluster was involved in “retrograde transport” (GO:0042147) and the orange cluster was related to “glutathione transferase activity” (GO:0004364).
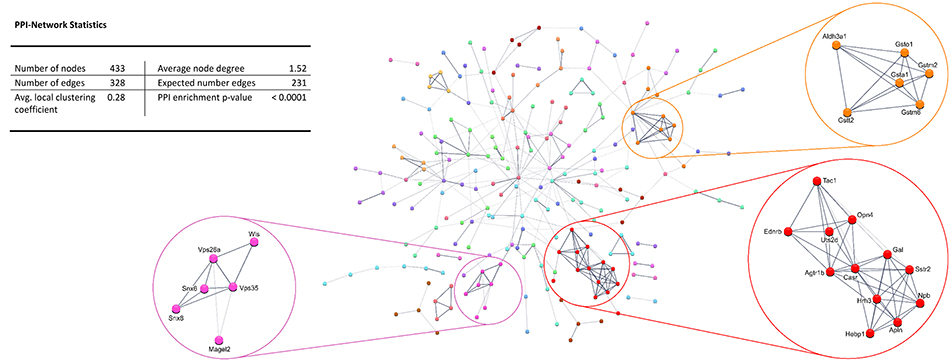
Figure 3. STRING database protein-protein interaction (PPI) network of significantly upregulated genes. Cut-off values, p ≤ 0.01, fold change ≥ 1.2.
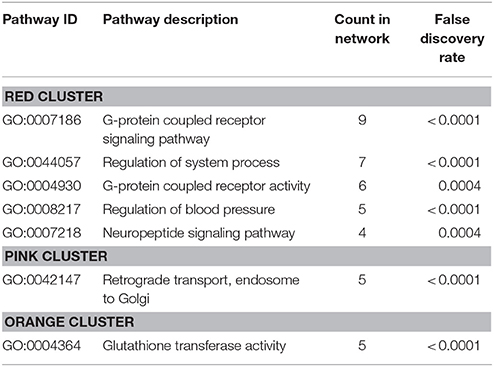
Table 4. GO biological processes and molecular functions of PPI-clusters from upregulated mRNAs in α-Gal A(−/0) vs. wildtype mice.
Enriched Pathways and Protein-Protein Interactions for Downregulated mRNAs
Enrichment analysis for the 306 significantly downregulated genes revealed a variety of regulated pathways, including immune related pathways (e.g., Complement and coagulation cascades, Antigen processing and presentation, Immune system process, Immune responses, etc.), autoimmune diseases (e.g., Systemic lupus erythematosus, Diabetes mellitus Type 1, Autoimmune thyroid disease, Asthma, etc.) and different infection pathways (e.g., Herpes simplex, Staphylococcus aureus, Leishmaniasis, etc.). In addition, “Neuroactive ligand-receptor interaction” (KEGG:04080) and “Vesicle” (GO:0031982) were enriched in the downregulated mRNAs (Table 5).
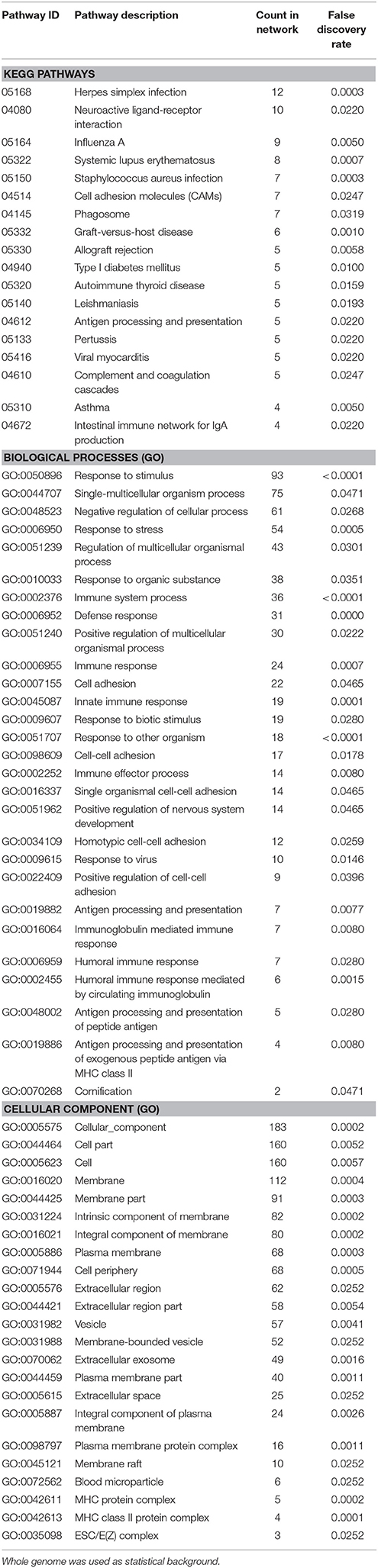
Table 5. Enrichment-analysis for downregulated mRNAs in α-Gal A(−/0) vs. wildtype mice using gene ontology and KEGG pathway annotations.
Also, the PPI analysis of significantly downregulated mRNAs revealed a significant PPI enrichment (p < 0.0001; Figure 4). Actually observed edges (n = 250) exceeded the expected number of edges (n = 134) by 87%. Also for the downregulated mRNAs clusters of interconnected proteins emerged. Enrichment analysis showed three clusters (i.e., green, purple, and cyan) related to the immune system (e.g., Immune system process—GO: 0002376, Immune response—GO:0006955). The blue cluster was associated with gene regulation (e.g., Chromatin modification—GO:0016568) and the rose cluster was related to “G-protein coupled receptor activity” (GO:0004930) (Table 6).
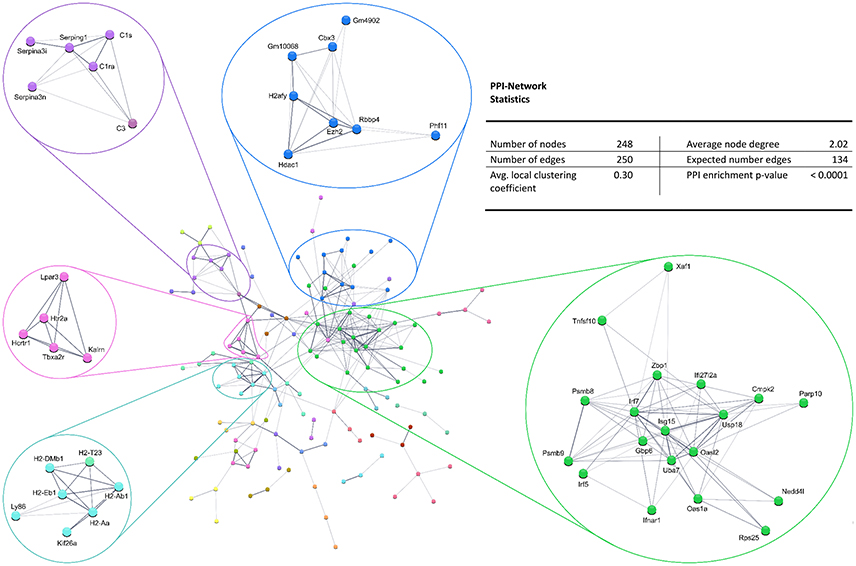
Figure 4. STRING database protein–protein interaction (PPI) network of significantly downregulated genes. Cut-off values, p ≤ 0.01, fold change ≥ 1.2.
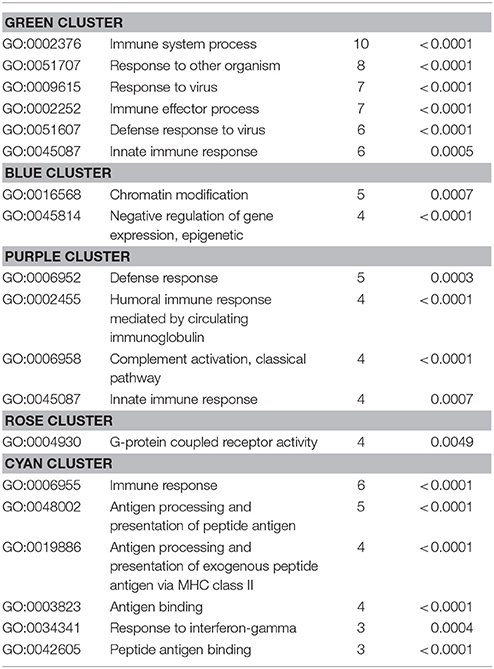
Table 6. GO biological processes and molecular functions of PPI-clusters from downregulated mRNAs in α-Gal A(−/0) vs. wildtype mice.
Ion Channel Regulation
As the sensory deficits of Fabry patients are generally accepted to be caused by changes in the excitability of sensory neurons, we specifically searched our dataset for genes related to ion channels, ion channel function and trafficking. Besides downregulation of voltage-gated sodium and calcium channels (i.e., Scn7a and Cacna1h), we found that several potassium channels and potassium channel associated proteins were differentially expressed (Table 7). Voltage-gated (i.e., Kcnb2) and calcium activated potassium channel subunits (i.e., Kcnmb1 and Kcnt1), as well as potassium channel tetramerization and interacting proteins (i.e., Kcnip2, Pctd16, and Kctd11) were downregulated in DRGs from FD mice. In contrast, the potassium channel ancillary beta subunit Kcne3 was upregulated. Last but not least, the mechanosensitive ion channel Piezo2 was significantly downregulated. Against all expectation, we found none of the pain-associated transient receptor potential (TRP) channels regulated.
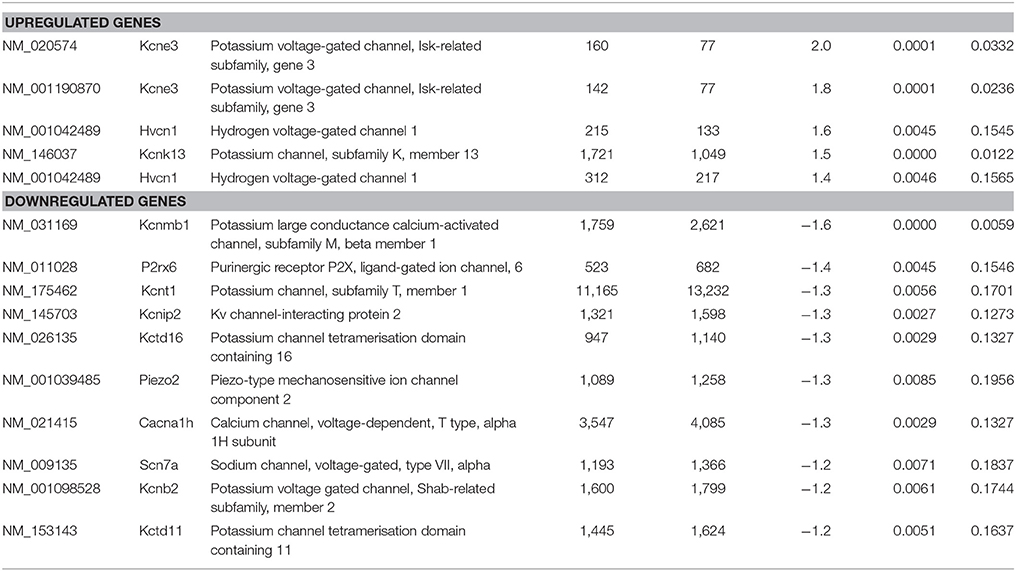
Table 7. Raw expression values, fold changes, and statistical analysis for significantly regulated ion channels.
RT-qPCR Validation of Regulated Genes
To validate the differentially expressed genes from the microarray expression profiling, we performed RT-qPCR analysis of the top 10 up- and downregulated genes in a separate set of samples from α-Gal A(−/0) mice backcrossed with C57BL/6J mice and C57BL/6J wildtype mice. We found that 9/10 of the upregulated genes (i.e., Rnf39, Opn4, Ecel1, Dnah8, Amz1, Dnase1l3, Meig1, Atf3, and Ncapg2) showed significant upregulation, whereas only one gene (i.e., Samd8) was not regulated (Figure 5A). For the downregulated genes, 6/10 genes (i.e., Slc51a, Zfp932, Gm1987, Syt15, Nnat, and Hdac1) were significantly downregulated, and four genes (i.e., Tmem25, S100pbp, Pmepa1, and Dock4) did not show regulation (Figure 5B). Thus, differential expression of 75% of the genes selected for RT-qPCR validation could be verified.
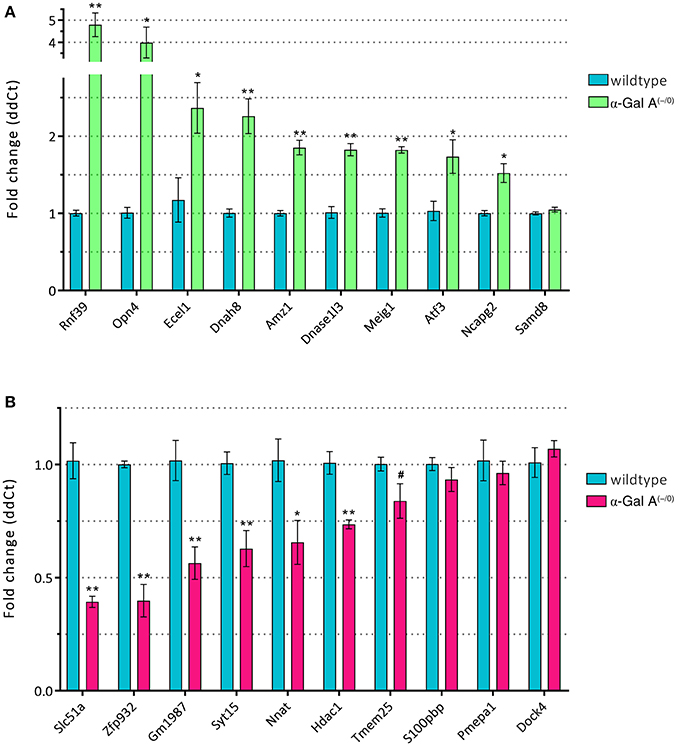
Figure 5. RT-qPCR validation of up- (A) and downregulated genes (B). *p < 0.05, **p < 0.01, #p < 0.1.
Discussion
Neuropathic pain and small-nerve fiber neuropathy are among the first symptoms of Fabry disease and affect the majority of patients already in early childhood. Therefore, the involvement of sensory neurons, whose cell somata are located in DRGs, is evident. However, our study is the first to present a differential gene expression profile of DRGs from α-Gal A(−/0) mice, a recognized mouse model for FD (Ohshima et al., 1997; Lakoma et al., 2014; Uceyler et al., 2016), and wildtype controls. We performed microarray expression profiling and found that 812 genes were significantly deregulated, 506 of them being upregulated and 306 being downregulated. Enrichment analysis revealed that the two pathways “lysosome” and “ceramide metabolic process” were significantly enriched. As FD is part of the broad family of lysosomal storage disorders that all show defects in ceramide metabolism (Platt et al., 2012), our results demonstrate the involvement of these two pathways also in DRG neurons and therefore enhance the reliability of the current analysis.
When taking a closer look at the significantly downregulated genes the “immune system” emerged as another disease specific entity. Lysosomal storage disorders in general are associated with deficits in processing of protein antigens and antibody production (Daly et al., 2000), and Hawkins-Salsbury et al. (2011) specifically report an immune deficit in Fabry patients. In the present study, enrichment analysis of downregulated genes revealed mainly immune system related pathways and processes, for example different autoimmune diseases, infection pathways and processes like “immune responses” or “antigen processing and presentation” (Table 5). In this regard, it might be noted that the downregulated purple cluster which includes serine-protease inhibitors (Serpins) might also be involved in nervous system related symptoms. Serpins are known to play a role in coagulation, and loss of serpins might induce a variety of bleeding disorders (Kaiserman et al., 2006). It has recently been shown that angiokeratoma, one of the first dermatologic disease presentations in Fabry patients, if present in gastrointestinal mucosa can lead to life-threatening bleeding episodes during coagulation therapy (Oh et al., 2016; Kang et al., 2017). Interestingly, 30% of Fabry patients show cerebral microbleeds (Kono et al., 2016), which together with the downregulated Thrombospondin 1 (Thsd7a) and Thromboxane a2 receptor (Tbxa2r) can be related to a general deficit in blood coagulation pathways. Further analysis of the regulation and impairment of those genes might open up new treatment strategies for cerebral vasculopathy, including cerebral hemorrhage, stroke, or other cerebral lesions associated with FD (Schiffmann and Moore, 2006). In a mouse model of neuropathic pain, it has been shown that mice that underwent surgery for chronic constriction injury showed activation of the immune system in higher brain structures (Koks et al., 2008). Based on these results it would be interesting to see if this immune activation is also present in brains of FD mice and/or patients.
Enrichment analysis of the upregulated clusters revealed significant enrichment of the “G-protein coupled receptor signaling” and “retrograde transport” pathways. Upregulation of genes in these clusters could be related to hypersensitivity and changes in excitability of DRG nerve fibers as a possible underlying cause of the frequent pain attacks experienced by Fabry patients (Schiffmann and Moore, 2006; Uceyler et al., 2014). Although the genes in the reported clusters are not directly related to changes in excitability, a number of ion channels were significantly deregulated and could be responsible for the hyperexcitability. Besides downregulation of voltage-gated sodium and calcium channels, different potassium channels and associated proteins showed regulation. In contrast, only Kcne3—a potassium channel ancillary beta subunit known to increase excitability (Abbott et al., 2001)—was upregulated. To date, knowledge on changes in ion channel expression and function in FD are sparse and controversial. Lakoma et al. (2014) reported increased immunoreactivity for a voltage-gated sodium channel Nav1.8 (Scn10a) in skin samples of FD mouse sensory neurons including the free nerve endings. Recently, decreased conductance of sodium currents in dissociated DRG neurons from FD mice was demonstrated (Namer et al., 2017). This latter publication also reported activation of voltage-gated potassium channels at more depolarized potentials, supporting a general reduction in FD neuron excitability (Namer et al., 2017). With regard to calcium channels it has been shown that Lyso-Gb3 enhances voltage-gated calcium currents in DRGs of FD mice (Choi L. et al., 2015), whereas Namer et al. (2017) report decreased voltage-gated calcium currents in α-Gal A(−/0) nociceptors. We also found a downregulation of the mechanosensitive ion channel Piezo2 mRNA, which may possibly be correlated to the decreased number of mechanosensitive fibers found in both human patients and FD mice (Namer et al., 2017). With regards to temperature sensitive ion channels it has been shown that expression of Trpv1 was increased, whereas expression of Trpm8 was decreased in skin biopsies of FD mice (Lakoma et al., 2014, 2016), which may be related to the changed thermal thresholds reported in both Fabry patients and mice (Sheth and Swick, 1980; Dutsch et al., 2002; Uceyler et al., 2013; Namer et al., 2017). The present unbiased screen for differentially expressed ion channels did not confirm deregulation of Trpv1 or Trpm8 though.
Previous gene expression studies were not performed in neuronal tissues but could still be affected by the same regulating pathways. In α-Gal A(−/0) fibroblasts and endothelial cells KCa3.1 (Kcnn4) was downregulated (Choi et al., 2014; Choi J. Y. et al., 2015) and the conductance of calcium-activated potassium channels was reduced (Olivan-Viguera et al., 2017). Additional gene expression studies have been performed in hepatic, renal and human blood cells (Park et al., 2009; Cigna et al., 2013; Shin et al., 2015). Thrombospondin 2 and 4 have been found to be upregulated in FD kidney cells (Park et al., 2009), whereas our results show a downregulation of both Thrombospondin 1 (Thsd7a) and Thromboxane a2 receptor (Tbxa2r) in FD DRGs. Both observations point towards impaired blood coagulation pathways in FD. In the same screen Neuropeptide Y (NPY) was found to be upregulated (Park et al., 2009). In the current dataset, a different neuropeptide, Neuropeptide B, was significantly upregulated, which has been shown to be functionally connected with NPY at least in fish (Yang et al., 2014). Furthermore, different types of S100 calcium binding proteins, i.e., S100a4/a8/a9 are upregulated in liver and kidney (Park et al., 2009), whereas S100pbp (a S100P binding protein) was decreased in FD DRGs in the present screen.
The deregulated genes that emerged from our analysis largely overlap with genes from previous reports on other painful disorders, although the direction of regulation does not always match. Upregulation of the transcription factor Atf3 is in line with previous reports that showed induction of Atf3 in DRGs in different models of nerve injury (Tsujino et al., 2000; Shortland et al., 2006; Matsuura et al., 2013), as well as upon exposure to noxious stimuli (Braz and Basbaum, 2010). Also, the adenosine receptor Adora2b which was upregulated in FD mice promotes chronic pain through neuro-immune interactions (Hu et al., 2016). The Tyrp1 gene has been associated with thermal nociception, and loss of function mutations generate deficits in thermal nociception (Fortin et al., 2010). Furthermore, Cdk5-mediated phosphorylation modulates Trpv1 function (Jendryke et al., 2016). Upregulation of these two latter genes in FD may therefore be associated with burning and tingling paraesthesias reported in Fabry patients (Germain, 2010; Ginsberg, 2013). Neuronatin (Nnat), which is significantly downregulated in the current screen, was upregulated in DRGs after sciatic nerve injury and associated with mechanical hypersensitivity (Chen et al., 2010). Several genes in the clusters that emerged from the current analysis, are associated with G-protein signaling and are controversially discussed (Pan et al., 2008). The somatostatin receptor Sstr2 in the red cluster is downregulated after sciatic nerve ligation (Shi et al., 2014), but elevated in response to intestinal inflammation (Van Op den Bosch et al., 2009). The endothelin receptor Ednrb attenuates cancer-induced pain (Viet et al., 2011), and the angiotensin receptor Agtr1b has been proposed as a biomarker for pain (Grace et al., 2012). All clusters involve genes that have been associated with exacerbated pain phenotypes in clinical or preclinical studies. Single nucleotide polymorphisms in the serotonin receptor gene Htr2a in the rose gene cluster are associated with pain-phenotypes as a genetic predisposition to musculoskeletal pain (Nicholl et al., 2011). The hypocretin receptor Hcrtr1 is associated with migraine (Rainero et al., 2011), and Kalirin (Klrn), a Rho guanine nucleotide exchange factor, is required for persistent nociceptive activity dependent synaptic long-term potentiation (Lu et al., 2015). The pink cluster contains genes that are mainly associated with retrograde transport. Vps26a is increased following spinal nerve ligation in the spinal dorsal horn and is required for recycling of mGluR5 and plasticity at excitatory synapses (Lin et al., 2015). Vps35, another regulated gene product from our screen, forms a complex with Vps26a (Kim et al., 2010) and is also highly associated with members from the sorting nexin family (e.g., Snx6 and Snx8 from our screen). Individuals with polymorphisms in Gluthatione-S-transferase genes found in the orange gene cluster are more likely to develop neuropathy during oxaliplatin treatment (Kanat et al., 2017). In addition, activation of Aldh2, a gene associated with the glutathione pathway, reduces nociception in acute inflammatory pain (Zambelli et al., 2014). This gene is regulated by Aldh3a1 which was deregulated in the current analysis (Chen et al., 2015). The green cluster contained the gene Tnfsf10, a member of the Tumor necrosis factor superfamily. Tnfsf10 is increased by excitotoxic spinal cord injury (Plunkett et al., 2001), downregulated in inflamed tissue (Yang et al., 2007), and associated with migraine susceptibility (Jia et al., 2015). Parp10, a poly(ADP-ribose) polymerase upregulates pro-inflammatory pathways, and its inhibition attenuates neuropathy and neuroinflammation (Komirishetty et al., 2016a,b). Interferon regulatory factor Irf5 is increased in spinal microglia after peripheral nerve injury and drives P2X4R+ reactive microglia thereby gating neuropathic pain (Masuda et al., 2014). In Chronic atypical neutrophilic dermatosis with lipodystrophy and elevated temperature (CANDLE), a disease exhibiting joint pain symptoms, mutations have been found in proteasome subunit genes Psmb8 and Psmb9 (Arimochi et al., 2016). Genes from the Oas dsRNA sensor family, in particular Oas1a and Oasl2, are induced by lipopolysaccharides, which induce inflammatory pain (Lee et al., 2013). The E3 ubiquitin ligase Nedd4 is decreased in DRGs of SNI mice (Laedermann et al., 2013), and ribosomal protein Rps25, as well as other ribosomal proteins are downregulated in a model for HIV-associated neuropathic pain (Maratou et al., 2009). In line with the downregulation of Hdac1 in the blue cluster, HDAC inhibitors attenuate the development of hypersensitivity (Denk et al., 2013), restore C-fiber sensitivity (Matsushita et al., 2013), and induce behavioral anti-nociception (Tao et al., 2016). In addition, nerve injury increases the activity of Hdac1 and Ezh2 (Laumet et al., 2015). Pain responses depend on genes from the major histocompatibility complex (MHC; Guo et al., 2015), and the MHC-2 haplotype is involved in the incidence of postherpetic pain (Sato-Takeda et al., 2006). Further, MHC-2 molecules synergize with Toll-like receptor Tlr4 in inducing an innate immune response (Frei et al., 2010), and the lymphocyte antigen Ly86 is required in DRG neurons for functional Toll-like receptor Tlr4 signaling (Grace et al., 2014). Serpina3n is upregulated in mouse DRGs following nerve injury and attenuates neuropathic pain. Mice lacking Serpina3n develop more severe neuropathic pain symptoms than wildtypes (Vicuna et al., 2015). Another member of the Serpin family—Serping—has been implicated in hereditary angioedema, as mutations in this gene are associated with abdominal pain symptoms (Andrejevic et al., 2015). Finally, the Complement component genes C1r, C1s and C3 are upregulated after spinal nerve ligation (Levin et al., 2008). When comparing the emerging FD pain related genes with the global pain systems network for heat nociception (Neely et al., 2012) only epidermal growth factor receptor pathway substrate 8 (Eps8), alpha-N-acetylgalactosaminidase (Naga) and the proteasome subunit gene Psmb8 were contained. Together, this comprehensive literature search demonstrates considerable overlap of the current FD expression profile with genes implicated in nociception and pain disorders, suggesting relevant common pathogenesis components of FD pain and other pain disorders.
Despite constituting the first presentation of differentially expressed genes in DRG explants of α-Gal A(−/0) mice, some limitations of the current analysis need to be considered. For instance, concerns have been raised that the α-Gal A(−/0) mouse model might only resemble the later-onset phenotype of FD (Bangari et al., 2015). In kidney, Gb3 concentrations only reach 25% of that found in patients, and FD mouse life expectancy is normal (Taguchi et al., 2013). Therefore, the G3Stg/GLA knockout mouse has been generated and evaluated as a new FD mouse model, in which α-Gal A(−/0) mice were crossbred with transgenic mice expressing the human Gb3 synthase. This resulted in symptomatic animals with increased Gb3 accumulation and progressive renal impairment (Taguchi et al., 2013). Another FD mouse model is the NOD/SCID immune deficiency mouse model with tissue specific Gb3 accumulation, but without clinical manifestation (Pacienza et al., 2012). Few data are available for these genetic models yet, but it would be important to know to what extent the three FD mouse models share the same differential gene expression. In addition, it would be of interest to explore the deregulation of gene expression in heterozygote females, which in humans and mice exhibit a considerably weaker phenotype than males (Uceyler et al., 2013, 2016). Screening of homozygote females could be helpful to better understand the mechanisms and degree of X-chromosomal inactivation in female Fabry patients (Wilcox et al., 2008). Finally, it should be noted that gene targeting experiments are prone to a general phenomenon of background dependence that might confound the interpretation of results (Schalkwyk et al., 2007). In this study we controlled for this effect by using α-Gal A(−/0) mice that had been backcrossed to an inbred C57BL/6J colony for the RT-qPCR validation of regulated genes.
Our in-depth bioinformatics analysis revealed a new set of genes and pathways that might be involved in the FD-associated small-nerve fiber neuropathy. These data give rise to subsequent functional studies on the importance of these deregulated genes for the pathogenesis of FD small fiber disease and neuropathic pain, and are expected to lead to the identification of novel treatment strategies, especially for neuropathic pain related symptoms in Fabry patients.
Author Contributions
KK, MK, and ML: designed the study; KK, TK, and ML: performed the data collection, analyzed, and interpreted the data; KK: wrote the manuscript. TK, MK, and ML: critically reviewed the contents of the paper and suggested substantial improvements; All authors have approved the final version of the manuscript.
Conflict of Interest Statement
The authors declare that the research was conducted in the absence of any commercial or financial relationships that could be construed as a potential conflict of interest.
Acknowledgments
The study was supported by the intramural MUI Start funding program for young scientists of the Medical University of Innsbruck (project number 2013042009, to ML) and an Austrian Science Fund (FWF) grant (project number ZFP253450, to MK).
References
Abbott, G. W., Butler, M. H., Bendahhou, S., Dalakas, M. C., Ptacek, L. J., and Goldstein, S. A. (2001). MiRP2 forms potassium channels in skeletal muscle with Kv3.4 and is associated with periodic paralysis. Cell 104, 217–231. doi: 10.1016/S0092-8674(01)00207-0
Andrejevic, S., Korosec, P., Silar, M., Kosnik, M., Mijanovic, R., Bonaci-Nikolic, B., et al. (2015). Hereditary angioedema due to C1 inhibitor deficiency in serbia: two novel mutations and evidence of genotype-phenotype association. PLoS ONE 10:e0142174. doi: 10.1371/journal.pone.0142174
Arimochi, H., Sasaki, Y., Kitamura, A., and Yasutomo, K. (2016). Differentiation of preadipocytes and mature adipocytes requires PSMB8. Sci. Rep. 6:26791. doi: 10.1038/srep26791
Bangari, D. S., Ashe, K. M., Desnick, R. J., Maloney, C., Lydon, J., Piepenhagen, P., et al. (2015). alpha-Galactosidase A knockout mice: progressive organ pathology resembles the type 2 later-onset phenotype of Fabry disease. Am. J. Pathol. 185, 651–665. doi: 10.1016/j.ajpath.2014.11.004
Benjamini, Y., and Hochberg, Y. (1995). Controlling the false discovery rate - a practical and powerful approach to multiple testing. J. R. Stat. Soc. Ser. B Methodol. 57, 289–300.
Braz, J. M., and Basbaum, A. I. (2010). Differential ATF3 expression in dorsal root ganglion neurons reveals the profile of primary afferents engaged by diverse noxious chemical stimuli. Pain 150, 290–301. doi: 10.1016/j.pain.2010.05.005
Chen, C. H., Cruz, L. A., and Mochly-Rosen, D. (2015). Pharmacological recruitment of aldehyde dehydrogenase 3A1 (ALDH3A1) to assist ALDH2 in acetaldehyde and ethanol metabolism in vivo. Proc. Natl. Acad. Sci. U.S.A. 112, 3074–3079. doi: 10.1073/pnas.1414657112
Chen, K. H., Yang, C. H., Cheng, J. T., Wu, C. H., Sy, W. D., and Lin, C. R. (2010). Altered neuronatin expression in the rat dorsal root ganglion after sciatic nerve transection. J. Biomed. Sci. 17:41. doi: 10.1186/1423-0127-17-41
Choi, J. Y., Shin, M. Y., Suh, S. H., and Park, S. (2015). Lyso-globotriaosylceramide downregulates KCa3.1 channel expression to inhibit collagen synthesis in fibroblasts. Biochem. Biophys. Res. Commun. 468, 883–888. doi: 10.1016/j.bbrc.2015.11.050
Choi, L., Vernon, J., Kopach, O., Minett, M. S., Mills, K., Clayton, P. T., et al. (2015). The Fabry disease-associated lipid Lyso-Gb3 enhances voltage-gated calcium currents in sensory neurons and causes pain. Neurosci. Lett. 594, 163–168. doi: 10.1016/j.neulet.2015.01.084
Choi, S., Kim, J. A., Na, H. Y., Cho, S. E., Park, S., Jung, S. C., et al. (2014). Globotriaosylceramide induces lysosomal degradation of endothelial KCa3.1 in fabry disease. Arterioscler. Thromb. Vasc. Biol. 34, 81–89. doi: 10.1161/ATVBAHA.113.302200
Cigna, D., D'anna, C., Zizzo, C., Francofonte, D., Sorrentino, I., Colomba, P., et al. (2013). Alteration of proteomic profiles in PBMC isolated from patients with Fabry disease: preliminary findings. Mol. Biosyst. 9, 1162–1168. doi: 10.1039/c3mb25402j
Daly, T. M., Lorenz, R. G., and Sands, M. S. (2000). Abnormal immune function in vivo in a murine model of lysosomal storage disease. Pediatr. Res. 47, 757–762. doi: 10.1203/00006450-200006000-00012
Denk, F., Huang, W., Sidders, B., Bithell, A., Crow, M., Grist, J., et al. (2013). HDAC inhibitors attenuate the development of hypersensitivity in models of neuropathic pain. Pain 154, 1668–1679. doi: 10.1016/j.pain.2013.05.021
Desnick, R. J., Ioannou, Y. A., and Eng, C. M. (2001). “Alpha-galactosidase A deficiency: Fabry disease,” in The Metabolic and Molecular Bases of Inherited Disease, eds C. R. Scriver, A. L. Beaudet, W. S. Sly, and D. Valle (New York, NY: McGraw-Hill), 3733–3774.
Dutsch, M., Marthol, H., Stemper, B., Brys, M., Haendl, T., and Hilz, M. J. (2002). Small fiber dysfunction predominates in Fabry neuropathy. J. Clin. Neurophysiol. 19, 575–586. doi: 10.1097/00004691-200212000-00011
Edgar, R., Domrachev, M., and Lash, A. E. (2002). Gene Expression Omnibus: NCBI gene expression and hybridization array data repository. Nucleic Acids Res. 30, 207–210. doi: 10.1093/nar/30.1.207
Fortin, A., Diez, E., Ritchie, J., Sotocinal, S. G., Dube, M. P., Gagne, M., et al. (2010). Positional cloning of a quantitative trait locus contributing to pain sensitivity: possible mediation by Tyrp1. Genes Brain Behav. 9, 856–867. doi: 10.1111/j.1601-183X.2010.00618.x
Frei, R., Steinle, J., Birchler, T., Loeliger, S., Roduit, C., Steinhoff, D., et al. (2010). MHC class II molecules enhance Toll-like receptor mediated innate immune responses. PLoS ONE 5:e8808. doi: 10.1371/journal.pone.0008808
Gal, A., Schafer, E., and Rohard, I. (2006). “Chapter 22: The genetic basis of Fabry disease,” in Fabry Disease: Perspectives from 5 Years of FOS, eds A. Mehta, M. Beck, and G. Sunder-Plassmann (Oxford: Oxford PharmaGenesis).
Ginsberg, L. (2013). “Monogenic disorder: Fabry disease,” in Stroke Genetics, eds S. Pankaj and J. F. Meschia (London: Springer), 97–106.
Godel, T., Baumer, P., Pham, M., Kohn, A., Muschol, N., Kronlage, M., et al. (2017). Human dorsal root ganglion in vivo morphometry and perfusion in Fabry painful neuropathy. Neurology 89, 1274–1282. doi: 10.1212/WNL.0000000000004396
Grace, P. M., Hurley, D., Barratt, D. T., Tsykin, A., Watkins, L. R., Rolan, P. E., et al. (2012). Harnessing pain heterogeneity and RNA transcriptome to identify blood-based pain biomarkers: a novel correlational study design and bioinformatics approach in a graded chronic constriction injury model. J. Neurochem. 122, 976–994. doi: 10.1111/j.1471-4159.2012.07833.x
Grace, P. M., Hutchinson, M. R., Maier, S. F., and Watkins, L. R. (2014). Pathological pain and the neuroimmune interface. Nat. Rev. Immunol. 14, 217–231. doi: 10.1038/nri3621
Guo, Y., Yao, F. R., Cao, D. Y., Li, L., Wang, H. S., Xie, W., et al. (2015). The major histocompatibility complex genes impact pain response in DA and DA.1U rats. Physiol. Behav. 147, 30–37. doi: 10.1016/j.physbeh.2015.04.009
Hawkins-Salsbury, J. A., Reddy, A. S., and Sands, M. S. (2011). Combination therapies for lysosomal storage disease: is the whole greater than the sum of its parts? Hum. Mol. Genet. 20, R54–R60. doi: 10.1093/hmg/ddr112
Hu, X., Adebiyi, M. G., Luo, J., Sun, K., Le, T. T., Zhang, Y., et al. (2016). Sustained elevated adenosine via ADORA2B promotes chronic pain through neuro-immune interaction. Cell Rep. 16, 106–119. doi: 10.1016/j.celrep.2016.05.080
Jendryke, T., Prochazkova, M., Hall, B. E., Nordmann, G. C., Schladt, M., Milenkovic, V. M., et al. (2016). TRPV1 function is modulated by Cdk5-mediated phosphorylation: insights into the molecular mechanism of nociception. Sci. Rep. 6:22007. doi: 10.1038/srep22007
Jia, S., Dong, W., Zhou, X., Chen, Z., and Yun, W. (2015). Association between TNFSF10 polymorphism and migraine susceptibility in a Chinese population. J. Int. Med. Res. 43, 326–331. doi: 10.1177/0300060514565681
Kaiserman, D., Whisstock, J. C., and Bird, P. I. (2006). Mechanisms of serpin dysfunction in disease. Expert Rev. Mol. Med. 8, 1–19. doi: 10.1017/S1462399406000184
Kanat, O., Ertas, H., and Caner, B. (2017). Platinum-induced neurotoxicity: a review of possible mechanisms. World J. Clin. Oncol. 8, 329–335. doi: 10.5306/wjco.v8.i4.329
Kang, E., Kim, Y. M., Kim, D. H., Yoo, H. W., and Lee, B. H. (2017). Life-threatening bleeding from gastric mucosal angiokeratomas during anticoagulation: a case report of Fabry disease. Medicine 96:e6063. doi: 10.1097/MD.0000000000006063
Kim, E., Lee, Y., Lee, H. J., Kim, J. S., Song, B. S., Huh, J. W., et al. (2010). Implication of mouse Vps26b-Vps29-Vps35 retromer complex in sortilin trafficking. Biochem. Biophys. Res. Commun. 403, 167–171. doi: 10.1016/j.bbrc.2010.10.121
Kocen, R. S., and Thomas, P. K. (1970). Peripheral Nerve Involvement in Fabrys Disease. Arch. Neurol. 22, 81–88. doi: 10.1001/archneur.1970.00480190085014
Koks, S., Fernandes, C., Kurrikoff, K., Vasar, E., and Schalkwyk, L. C. (2008). Gene expression profiling reveals upregulation of Tlr4 receptors in Cckb receptor deficient mice. Behav. Brain Res. 188, 62–70. doi: 10.1016/j.bbr.2007.10.020
Komirishetty, P., Areti, A., Gogoi, R., Sistla, R., and Kumar, A. (2016a). Poly(ADP-ribose) polymerase inhibition reveals a potential mechanism to promote neuroprotection and treat neuropathic pain. Neural Regen. Res. 11, 1545–1548. doi: 10.4103/1673-5374.193222
Komirishetty, P., Areti, A., Yerra, V. G., Ruby, P. K., Sharma, S. S., Gogoi, R., et al. (2016b). PARP inhibition attenuates neuroinflammation and oxidative stress in chronic constriction injury induced peripheral neuropathy. Life Sci. 150, 50–60. doi: 10.1016/j.lfs.2016.02.085
Kono, Y., Wakabayashi, T., Kobayashi, M., Ohashi, T., Eto, Y., Ida, H., et al. (2016). Characteristics of cerebral microbleeds in patients with Fabry disease. J. Stroke Cerebrovasc. Dis. 25, 1320–1325. doi: 10.1016/j.jstrokecerebrovasdis.2016.02.019
Laedermann, C. J., Cachemaille, M., Kirschmann, G., Pertin, M., Gosselin, R. D., Chang, I., et al. (2013). Dysregulation of voltage-gated sodium channels by ubiquitin ligase NEDD4-2 in neuropathic pain. J. Clin. Invest. 123, 3002–3013. doi: 10.1172/JCI68996
Lakoma, J., Rimondini, R., Donadio, V., Liguori, R., and Caprini, M. (2014). Pain related channels are differentially expressed in neuronal and non-neuronal cells of glabrous skin of fabry knockout male mice. PLoS ONE 9:e108641. doi: 10.1371/journal.pone.0108641
Lakoma, J., Rimondini, R., Ferrer Montiel, A., Donadio, V., Liguori, R., and Caprini, M. (2016). Increased expression of Trpv1 in peripheral terminals mediates thermal nociception in Fabry disease mouse model. Mol. Pain 12, 1–16. doi: 10.1177/1744806916663729
Langeslag, M., Malsch, P., Welling, A., and Kress, M. (2014). Reduced excitability of gp130-deficient nociceptors is associated with increased voltage-gated potassium currents and Kcna4 channel upregulation. Pflugers Arch. 466, 2153–2165. doi: 10.1007/s00424-014-1443-0
Laumet, G., Garriga, J., Chen, S. R., Zhang, Y., Li, D. P., Smith, T. M., et al. (2015). G9a is essential for epigenetic silencing of K(+) channel genes in acute-to-chronic pain transition. Nat. Neurosci. 18, 1746–1755. doi: 10.1038/nn.4165
Lee, M. S., Kim, B., Lee, S. M., Cho, W. C., Lee, W. B., Kang, J. S., et al. (2013). Genome-wide profiling of in vivo LPS-responsive genes in splenic myeloid cells. Mol. Cells 35, 498–513. doi: 10.1007/s10059-013-2349-y
Levin, M. E., Jin, J. G., Ji, R. R., Tong, J., Pomonis, J. D., Lavery, D. J., et al. (2008). Complement activation in the peripheral nervous system following the spinal nerve ligation model of neuropathic pain. Pain 137, 182–201. doi: 10.1016/j.pain.2007.11.005
Lin, T. B., Lai, C. Y., Hsieh, M. C., Wang, H. H., Cheng, J. K., Chau, Y. P., et al. (2015). VPS26A-SNX27 interaction-dependent mGluR5 recycling in dorsal horn neurons mediates neuropathic pain in rats. J. Neurosci. 35, 14943–14955. doi: 10.1523/JNEUROSCI.2587-15.2015
Lu, J., Luo, C., Bali, K. K., Xie, R. G., Mains, R. E., Eipper, B. A., et al. (2015). A role for Kalirin-7 in nociceptive sensitization via activity-dependent modulation of spinal synapses. Nat. Commun. 6:6820. doi: 10.1038/ncomms7820
Maratou, K., Wallace, V. C., Hasnie, F. S., Okuse, K., Hosseini, R., Jina, N., et al. (2009). Comparison of dorsal root ganglion gene expression in rat models of traumatic and HIV-associated neuropathic pain. Eur. J. Pain 13, 387–398. doi: 10.1016/j.ejpain.2008.05.011
Masuda, T., Iwamoto, S., Yoshinaga, R., Tozaki-Saitoh, H., Nishiyama, A., Mak, T. W., et al. (2014). Transcription factor IRF5 drives P2X4R+-reactive microglia gating neuropathic pain. Nat. Commun. 5, 3771. doi: 10.1038/ncomms4771
Matsushita, Y., Araki, K., Omotuyi, O., Mukae, T., and Ueda, H. (2013). HDAC inhibitors restore C-fibre sensitivity in experimental neuropathic pain model. Br. J. Pharmacol. 170, 991–998. doi: 10.1111/bph.12366
Matsuura, Y., Ohtori, S., Iwakura, N., Suzuki, T., Kuniyoshi, K., and Takahashi, K. (2013). Expression of activating transcription factor 3 (ATF3) in uninjured dorsal root ganglion neurons in a lower trunk avulsion pain model in rats. Eur. Spine J. 22, 1794–1799. doi: 10.1007/s00586-013-2733-5
Mechtler, T. P., Stary, S., Metz, T. F., De Jesus, V. R., Greber-Platzer, S., Pollak, A., et al. (2012). Neonatal screening for lysosomal storage disorders: feasibility and incidence from a nationwide study in Austria. Lancet 379, 335–341. doi: 10.1016/S0140-6736(11)61266-X
Namer, B., Orstavik, K., Schmidt, R., Mair, N., Kleggetveit, I. P., Zeidler, M., et al. (2017). Changes in ionic conductance signature of nociceptive neurons underlying Fabry disease phenotype. Front. Neurol. 8:335. doi: 10.3389/fneur.2017.00335
Neely, G. G., Rao, S., Costigan, M., Mair, N., Racz, I., Milinkeviciute, G., et al. (2012). Construction of a global pain systems network highlights phospholipid signaling as a regulator of heat nociception. PLoS Genet. 8:e1003071. doi: 10.1371/journal.pgen.1003071
Nicholl, B. I., Holliday, K. L., Macfarlane, G. J., Thomson, W., Davies, K. A., O'neill, T. W., et al. (2011). Association of HTR2A polymorphisms with chronic widespread pain and the extent of musculoskeletal pain: results from two population-based cohorts. Arthritis Rheum. 63, 810–818. doi: 10.1002/art.30185
Oh, H. J., Cho, Y. K., and Lee, H. H. (2016). Bleeding angiokeratomas in Fabry Disease treated with argon plasma coagulation. Clin. Gastroenterol. Hepatol. 14, e129–e130. doi: 10.1016/j.cgh.2016.03.042
Ohnishi, A., and Dyck, P. J. (1974). Loss of small peripheral sensory neurons in Fabry disease - histologic and morphometric evaluation of cutaneous nerves, spinal ganglia, and posterior columns. Arch. Neurol. 31, 120–127. doi: 10.1001/archneur.1974.00490380068009
Ohshima, T., Murray, G. J., Swaim, W. D., Longenecker, G., Quirk, J. M., Cardarelli, C. O., et al. (1997). alpha-Galactosidase A deficient mice: a model of Fabry disease. Proc. Natl. Acad. Sci. U.S.A. 94, 2540–2544. doi: 10.1073/pnas.94.6.2540
Olivan-Viguera, A., Lozano-Gerona, J., Lopez De Frutos, L., Cebolla, J. J., Irun, P., Abarca-Lachen, E., et al. (2017). Inhibition of intermediate-conductance calcium-activated K Channel (KCa3.1) and fibroblast mitogenesis by alpha-linolenic acid and alterations of channel expression in the lysosomal storage disorders, Fabry disease, and Niemann Pick C. Front. Physiol. 8:39. doi: 10.3389/fphys.2017.00039
Pacienza, N., Yoshimitsu, M., Mizue, N., Au, B. C., Wang, J. C., Fan, X., et al. (2012). Lentivector transduction improves outcomes over transplantation of human HSCs alone in NOD/SCID/Fabry mice. Mol. Ther. 20, 1454–1461. doi: 10.1038/mt.2012.64
Pan, H. L., Wu, Z. Z., Zhou, H. Y., Chen, S. R., Zhang, H. M., and Li, D. P. (2008). Modulation of pain transmission by G-protein-coupled receptors. Pharmacol. Ther. 117, 141–161. doi: 10.1016/j.pharmthera.2007.09.003
Park, E. S., Choi, J. O., Park, J. W., Lee, M. H., Park, H. Y., and Jung, S. C. (2009). Expression of genes and their responses to enzyme replacement therapy in a Fabry disease mouse model. Int. J. Mol. Med. 24, 401–407. doi: 10.3892/ijmm_00000246
Platt, F. M., Boland, B., and Van Der Spoel, A. C. (2012). The cell biology of disease: lysosomal storage disorders: the cellular impact of lysosomal dysfunction. J. Cell Biol. 199, 723–734. doi: 10.1083/jcb.201208152
Plunkett, J. A., Yu, C. G., Easton, J. M., Bethea, J. R., and Yezierski, R. P. (2001). Effects of interleukin-10 (IL-10) on pain behavior and gene expression following excitotoxic spinal cord injury in the rat. Exp. Neurol. 168, 144–154. doi: 10.1006/exnr.2000.7604
R Development Core Team (2008). R: A Language and Environment for Statistical Computing. Vienna: R Foundation for Statistical Computing.
Rainero, I., Rubino, E., Gallone, S., Fenoglio, P., Picci, L. R., Giobbe, L., et al. (2011). Evidence for an association between migraine and the hypocretin receptor 1 gene. J. Headache Pain 12, 193–199. doi: 10.1007/s10194-011-0314-8
Saito, S., Ohno, K., and Sakuraba, H. (2011). Fabry-database.org: database of the clinical phenotypes, genotypes and mutant alpha-galactosidase A structures in Fabry disease. J. Hum. Genet. 56, 467–468. doi: 10.1038/jhg.2011.31
Sato-Takeda, M., Takasaki, I., Takeda, K., Sasaki, A., Andoh, T., Nojima, H., et al. (2006). Major histocompatibility complex haplotype is associated with postherpetic pain in mice. Anesthesiology 104, 1063–1069. doi: 10.1097/00000542-200605000-00024
Schalkwyk, L. C., Fernandes, C., Nash, M. W., Kurrikoff, K., Vasar, E., and Koks, S. (2007). Interpretation of knockout experiments: the congenic footprint. Genes Brain Behav. 6, 299–303. doi: 10.1111/j.1601-183X.2007.00304.x
Schiffmann, R., and Moore, D. F. (2006). “Chapter 22: Neurological manifestations of Fabry disease,” in Fabry Disease: Perspectives from 5 Years of FOS, eds A. Mehta, M. Beck, and G. Sunder-Plassmann (Oxford: Oxford PharmaGenesis).
Sheth, K. J., and Swick, H. M. (1980). Peripheral nerve conduction in Fabry disease. Ann. Neurol. 7, 319–323. doi: 10.1002/ana.410070406
Shi, T. J., Xiang, Q., Zhang, M. D., Barde, S., Kai-Larsen, Y., Fried, K., et al. (2014). Somatostatin and its 2A receptor in dorsal root ganglia and dorsal horn of mouse and human: expression, trafficking and possible role in pain. Mol. Pain 10:12. doi: 10.1186/1744-8069-10-12
Shin, Y. J., Jeon, Y. J., Jung, N., Park, J. W., Park, H. Y., and Jung, S. C. (2015). Substrate-specific gene expression profiles in different kidney cell types are associated with Fabry disease. Mol. Med. Rep. 12, 5049–5057. doi: 10.3892/mmr.2015.4010
Shortland, P. J., Baytug, B., Krzyzanowska, A., McMahon, S. B., Priestley, J. V., and Averill, S. (2006). ATF3 expression in L4 dorsal root ganglion neurons after L5 spinal nerve transection. Eur. J. Neurosci. 23, 365–373. doi: 10.1111/j.1460-9568.2005.04568.x
Spada, M., Pagliardini, S., Yasuda, M., Tukel, T., Thiagarajan, G., Sakuraba, H., et al. (2006). High incidence of later-onset fabry disease revealed by newborn screening. Am. J. Hum. Genet. 79, 31–40. doi: 10.1086/504601
Szklarczyk, D., Morris, J. H., Cook, H., Kuhn, M., Wyder, S., Simonovic, M., et al. (2017). The STRING database in 2017: quality-controlled protein-protein association networks, made broadly accessible. Nucleic Acids Res. 45, D362–D368. doi: 10.1093/nar/gkw937
Taguchi, A., Maruyama, H., Nameta, M., Yamamoto, T., Matsuda, J., Kulkarni, A. B., et al. (2013). A symptomatic Fabry disease mouse model generated by inducing globotriaosylceramide synthesis. Biochem. J. 456, 373–383. doi: 10.1042/BJ20130825
Tao, W., Zhou, W., Wang, Y., Sun, T., Wang, H., Zhang, Z., et al. (2016). Histone deacetylase inhibitor-induced emergence of synaptic delta-opioid receptors and behavioral antinociception in persistent neuropathic pain. Neuroscience 339, 54–63. doi: 10.1016/j.neuroscience.2016.09.015
Tsujino, H., Kondo, E., Fukuoka, T., Dai, Y., Tokunaga, A., Miki, K., et al. (2000). Activating transcription factor 3 (ATF3) induction by axotomy in sensory and motoneurons: a novel neuronal marker of nerve injury. Mol. Cell. Neurosci. 15, 170–182. doi: 10.1006/mcne.1999.0814
Uceyler, N., Biko, L., Hose, D., Hofmann, L., and Sommer, C. (2016). Comprehensive and differential long-term characterization of the alpha-galactosidase A deficient mouse model of Fabry disease focusing on the sensory system and pain development. Mol. Pain 12, 1–10. doi: 10.1177/1744806916646379
Uceyler, N., Ganendiran, S., Kramer, D., and Sommer, C. (2014). Characterization of pain in fabry disease. Clin. J. Pain 30, 915–920. doi: 10.1097/AJP.0000000000000041
Uceyler, N., Kahn, A. K., Kramer, D., Zeller, D., Casanova-Molla, J., Wanner, C., et al. (2013). Impaired small fiber conduction in patients with Fabry disease: a neurophysiological case-control study. BMC Neurol. 13:47. doi: 10.1186/1471-2377-13-47
Van Op den Bosch, J., Van Nassauw, L., Van Marck, E., and Timmermans, J. P. (2009). Somatostatin modulates mast cell-induced responses in murine spinal neurons and satellite cells. Am. J. Physiol. Gastrointest. Liver Physiol. 297, G406–G417. doi: 10.1152/ajpgi.00059.2009
Vicuna, L., Strochlic, D. E., Latremoliere, A., Bali, K. K., Simonetti, M., Husainie, D., et al. (2015). The serine protease inhibitor SerpinA3N attenuates neuropathic pain by inhibiting T cell-derived leukocyte elastase. Nat. Med. 21, 518–523. doi: 10.1038/nm.3852
Viet, C. T., Ye, Y., Dang, D., Lam, D. K., Achdjian, S., Zhang, J., et al. (2011). Re-expression of the methylated EDNRB gene in oral squamous cell carcinoma attenuates cancer-induced pain. Pain 152, 2323–2332. doi: 10.1016/j.pain.2011.06.025
Wilcox, W. R., Oliveira, J. P., Hopkin, R. J., Ortiz, A., Banikazemi, M., Feldt-Rasmussen, U., et al. (2008). Females with Fabry disease frequently have major organ involvement: lessons from the Fabry Registry. Mol. Genet. Metab. 93, 112–128. doi: 10.1016/j.ymgme.2007.09.013
Yang, H. Y., Mitchell, K., Keller, J. M., and Iadarola, M. J. (2007). Peripheral inflammation increases Scya2 expression in sensory ganglia and cytokine and endothelial related gene expression in inflamed tissue. J. Neurochem. 103, 1628–1643. doi: 10.1111/j.1471-4159.2007.04874.x
Yang, L., Sun, C., and Li, W. (2014). Neuropeptide B in Nile tilapia Oreochromis niloticus: molecular cloning and its effects on the regulation of food intake and mRNA expression of growth hormone and prolactin. Gen. Comp. Endocrinol. 200, 27–34. doi: 10.1016/j.ygcen.2014.01.016
Keywords: Fabry disease, alpha Galactosidase A, lysosomal storage disorder, neuropathy, neurodegeneration, neuropathic pain
Citation: Kummer KK, Kalpachidou T, Kress M and Langeslag M (2018) Signatures of Altered Gene Expression in Dorsal Root Ganglia of a Fabry Disease Mouse Model. Front. Mol. Neurosci. 10:449. doi: 10.3389/fnmol.2017.00449
Received: 06 September 2017; Accepted: 22 December 2017;
Published: 25 January 2018.
Edited by:
Ildikó Rácz, Universitätsklinikum Bonn, GermanyReviewed by:
Pedro Brites, Instituto de Biologia Molecular e Celular (IBMC), PortugalSulev Kõks, University of Tartu, Estonia
Eldi Schonfeld-Dado, Stanford University, United States
Copyright © 2018 Kummer, Kalpachidou, Kress and Langeslag. This is an open-access article distributed under the terms of the Creative Commons Attribution License (CC BY). The use, distribution or reproduction in other forums is permitted, provided the original author(s) or licensor are credited and that the original publication in this journal is cited, in accordance with accepted academic practice. No use, distribution or reproduction is permitted which does not comply with these terms.
*Correspondence: Kai K. Kummer, kai.kummer@i-med.ac.at