Biology of Saccular Cerebral Aneurysms: A Review of Current Understanding and Future Directions
- Department of Neurosurgery, Barrow Neurological Institute, St. Joseph’s Hospital and Medical Center, Phoenix, AZ, USA
Understanding the biology of intracranial aneurysms is a clinical quandary. How these aneurysms form, progress, and rupture is poorly understood. Evidence indicates that well-established risk factors play a critical role, along with immunologic factors, in their development and clinical outcomes. Much of the expanding knowledge of the inception, progression, and rupture of intracranial aneurysms implicates inflammation as a critical mediator of aneurysm pathogenesis. Thus, therapeutic targets exploiting this arm of aneurysm pathogenesis have been implemented, often with promising outcomes.
Introduction
Intracranial aneurysms are common abnormalities of the brain (1–30). The reported prevalence was 3.2% in a homogeneous Finnish population and up to 5% in others (31, 32). The overall risk of rupture is about 1% (33, 34). At 40–65%, the overall lethality of subarachnoid hemorrhage (SAH) resulting from cerebral aneurysm rupture is significant (31, 35, 36). Thus, SAH remains a challenging clinical issue (31, 32, 37–43). Of patients who survive the initial ictus, ≤50% face significant morbidity (31, 38, 40, 44, 45).
The true natural history of cerebral aneurysms is incompletely understood. Types of cerebral aneurysms include giant, fusiform, and saccular. In this review, we focus on saccular aneurysms. Although much of the aneurysm biology remains unknown, a growing body of literature addresses their formation, progression, and rupture.
Clinical Risk Factors
Risk factors for intracranial aneurysms include the epidemiological risk factors of female sex, smoking, hypertension, and family history, which is the strongest indicator of rupture among non-modifiable risk factors. Compared to the general population, first-degree relatives of persons with intracranial aneurysms or previous SAH have a risk 3–7 times higher and tend to have ruptured aneurysms at younger ages than those with sporadic aneurysms (37, 38, 40, 42, 46–48). In a cohort of 142 patients with 181 unruptured aneurysms followed from the 1950s until 1997–1998 for death or SAH, the annual incidence of hemorrhage was 1.3% (36). Cumulative rates of bleeding were 11% at 10 years, 23% at 20 years, and 30% at 30 years. Associated risk factors were aneurysm diameter and age. Smoking was an independent covariate related to rupture risk.
Anatomical and Circulatory Factors
Aneurysms develop at branch points of high intravascular turbulence and abnormal vessel wall shear stress. They arise in areas with complex arterial vascular geometry, particularly bifurcations and curvatures that contribute to increases in wall shear stress. Although formation is linked to diffuse genetic/familial, environmental, and immunologic risk factors, saccular aneurysms seldom occur in random locations (31, 43). They tend to arise in sites similar to where giant and fusiform aneurysms form, with comparable and predictable geometric and anatomical properties. Vascular flow is turbulent or laminar. Turbulent flow has random variations in temporal and spatial components, with inconsistent predictability (43). Laminar flow typically occurs in large, straight vessels and is synonymous with normal physiological conditions but can be more complex or “disturbed,” occurring in areas of arterial bifurcations or poststenotic areas (49–52). These perturbations in flow often result in endothelial dysfunction, aiding aneurysm formation (31, 43). The endothelial response to wall shear stress appears to cause a cascade of gene signaling, morphological, and phenotypic changes that result in the initiation, progression, and rupture of intracranial aneurysms.
The locations of aneurysms are relatively consistent, with most cerebral aneurysms in the circle of Willis (43). However, considerable anatomical variability results from population-level differences in the individual geometry of the circle of Willis. Only 40% of people have a characteristic “complete” circle of Willis (43, 53). Unlike most large extracranial arteries, the bifurcation apex in cerebral vessels does not have consistent histologic media. Furthermore, the cerebral bifurcation apex has significantly less structural support from perivascular tissue (43, 54). Hemodynamic data suggest that deviations from optimal geometric constructs predispose specific vessels to aneurysm formation.
Approximately 90% of cerebral aneurysms occur in the anterior circulation, commonly (30–35%) the anterior communicating artery complex, followed by the internal carotid artery (30%) and associated branches (posterior communicating, ophthalmic arteries). Lastly, 22% occur in the middle cerebral artery and 10% in the posterior circulation (basilar apex, superior cerebellar artery, posterior inferior cerebellar artery) (40). These locations correlate with the distribution of intracranial atherosclerosis and areas of suboptimal hemodynamic patterns (40, 43). Known anatomical differences in familial aneurysms also account for approximately 10% of SAHs (38). Familial aneurysms typically are multiple and occur in the middle cerebral artery.
Aneurysm Formation and the Role of Inflammation
Numerous immunologic factors may influence the formation of intracranial aneurysms and their progression and rupture.
Pathology
The pathophysiological underpinnings of a saccular cerebral aneurysm may lie in an atherosclerotic pathway. Animal modeling points to damage of the internal elastic lamina that may define early aneurysm formation and change (55–60). Further atherosclerotic changes within the aneurysm wall are also described (61, 62). Structural differences occur in both small and large saccular aneurysms. Small aneurysms have diffuse intimal thickening, with proliferating vascular smooth muscle cells (VSMCs) and a preponderance of macrophages and lymphocytes. Larger aneurysms have more advanced atherosclerotic changes, particularly with phenotypic changes in VSMCs, lipid-laden macrophages, and lymphocytic infiltration.
Our current understanding of atherosclerosis as a contributor to cerebral aneurysm formation and progression is rooted in efforts to define abdominal aortic aneurysms (63–66). Individuals with both cerebral and abdominal aneurysms share comorbid risk factors, such as smoking and arterial hypertension. Immunologic response and chronic inflammation are key pathogenic features of atherosclerosis (67–73). These immunologic responses suggest that inflammatory mediators could be linked to the formation, progression, and rupture of cerebral aneurysms (31).
Vessel Wall Changes
Histologic changes in aneurysm formation include vessel wall damage as a precursor. Normal vessel walls are organized into distinct layers, while aneurysmal vessel walls have fewer distinct layers characterized by disintegration of the internal elastic lamina, progressive disorganization of the muscular media, intimal hyperplasia, and progressive irregularity of the luminal surface (74–79). Healthy cerebral vessels have a mix of collagen and connective tissue (type I, III, and IV), fibronectin, and laminin. Type I collagen exists mostly in adventitia and fibronectin in the media of normal vessels (80). However, vascular remodeling changes the vessel wall. Type I collagen increases and fibronectin is dispersed in the wall, while the levels of type III and IV collagen and laminin decrease (54).
Structural and pathological changes occur in the endothelium and VSMCs. Functioning vascular endothelium promotes vasodilation and is antiatherogenic; it also inhibits platelet adhesion and accumulation, VSMC proliferation and leukocyte adherence, and pro-inflammatory cascades. Recent evidence points to damage of the vascular endothelium as the inciting event, leading to the creation, inflammatory cascade, progression, and rupture of intracranial aneurysms (81–83). The key inciting event in endothelial injury may be hemodynamic stress (76).
Perturbations in the vascular endothelium appear constant in both experimental and human intracranial aneurysms (75, 77, 81, 84–89). Damage to the vascular endothelium incites morphologic and pathologic changes likely occurring in stages. The earliest changes (e.g., partial loss of endothelium) occur upon aneurysm formation and the latest (e.g., intimal swelling) upon progression. Initial morphologic and functional changes in the endothelium could be a response to shear stress. Endothelial cells become elongated and realign with directional blood flow. Changes also occur in the development of actin stress fibers that may alter endothelial cell density or migration (90, 91). Hemodynamic stress may alter acute and chronic inflammatory signaling pathways. Shear stress appears to activate mediating pathways of inflammation within endothelial cells [prostaglandin E(2)–E-prostanoid(2) (PGE(2)–EP(2))]. It also may amplify the chronic inflammatory pathway via nuclear factor-κB (92).
Changes in vessel walls are punctuated by changes in the vascular endothelium that occur in concert with phenotypic and morphologic changes in VSMCs supporting the media layer of the intracranial vasculature and providing structural support to vessel walls. Dynamic changes and eventual loss of the media layer contribute to aneurysm formation and rupture (80). Histologic evidence suggests that normally contractile VSMCs respond to environmental cues by undergoing phenotypic changes causing them to resemble a pro-inflammatory, pro-remodeling, and dedifferentiated phenotype (93–95). Normal differentiation of cerebral VSMCs includes high levels of largely contractile proteins comprising smooth muscle-myosin heavy chains, smooth muscle alpha-actin, and semicarbazide amine oxidase, which regulate VSMC differentiation (54, 96–105). An early morphologic finding was related to phenotypic changes in these proteins. The spindle-like VSMCs change into spider-like cells that migrated to and proliferated in the media, resulting in myointimal hyperplasia (99). These changes may be punctuated by the previously mentioned hemodynamic factors, macrophage and endothelial cell-derived mediators [tumor necrosis factor (TNF)-α, interleukin (IL)-β, nitric oxide, and growth factors], environmental factors, and genetic changes (54, 100, 102, 104). This punctuated VSMC transition results in proliferation of a pro-inflammatory phenotype of VSMCs. The pro-inflammatory phenotype is characterized by reduced levels of the contractile elements of VSMCs: smooth muscle-myosin heavy chains, smooth muscle alpha-actin, and semicarbazide-sensitive amine oxidase (54, 102). Further changes in the increase in transcription factors (Ets-1, p47phox, IL-6, monocyte chemoattractant protein-1, reactive oxygen species, matrix metalloproteinases, cathepsins), promoting inflammation, recruiting reactive oxygen species, and matrix remodeling, are identified as potentially promoting aneurysm progression (96, 98, 103, 106). Ultimately, these changes result in decreased expression of collagen biosynthesis and further loss of VSMCs, weakening the aneurysm wall and predisposing to aneurysm rupture (31).
Specific Inflammatory Pathways
The specific immunologic pathways and mediators involved in aneurysm formation remain partially understood. However, the immunologic effect can be divided into three areas linked to endothelial cells, VSMCs, and leukocytes. A common pathway for aneurysm formation is linked to certain leukocytes with distinct pathways of influence and known associated inflammatory mediators catalyzed by endothelial injury (31). The immunologic function is mediated by endothelial dysfunction, and the primary inflammatory mediators are NF-κB, Ets-1, MCP1, IL-1β, nitric oxide, angiotensin II, phosphodiesterase-4, and PGE(2)–EP(2) (Figure 1) (31). Dysfunctional major pathways of VSMCs include pro-inflammatory and pro-matrix remodeling, along with phenotypic modulation and associated apoptotic cell death. The major inflammatory mediators involved in VSMCs are IL-1β, p47phox, Ets-1, MCP1, angiotensin II, reactive oxygen species, matrix metalloproteinase, and cathepsins (31, 84, 107). Leukocytes, particularly mast cells and T-cells, influence aneurysm formation via a chronic inflammatory pathway associated with vessel wall remodeling and damage, with subsequent apoptotic cell death. Several inflammatory mediators are associated with leukocytes: TNF-α, IL-1β, IL-6, TLR4, Fas, nitric oxide, complement, IgG, IgM, basic fibroblast growth factor, TGF-α + β, vascular endothelial growth factor, reactive oxygen species, matrix metalloproteinases, and cathepsins (31, 108, 109). Understanding how these specific inflammatory mediators function opens the door to treatments targeting these major inflammatory pathways (31).
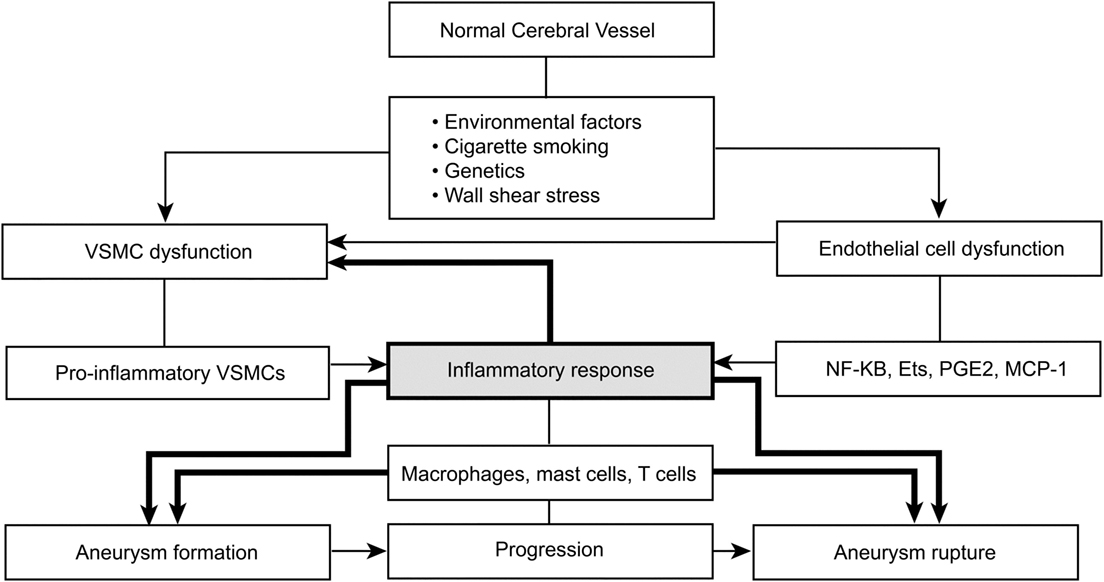
Figure 1. Environmental factors and immunologic pathways and mediators involved in aneurysm formation. Shading emphasizes the contribution of inflammation to the process of aneurysm formation. VSMC, vascular smooth muscle cell; NF-κB, nuclear factor-κB; Ets, E-twenty-six family transcription factors; PGE2, prostaglandin E2; MCP1, monocyte chemoattractant protein 1. Used with permission from Barrow Neurological Institute, Phoenix, AZ, USA.
Genetic Factors
Genetic factors contribute to the formation, progression, and rupture of intracranial aneurysms. Several studies have used microarray polymerase chain reaction to characterize the nature of these lesions. Despite further elucidating the gene expression profiles of these lesions, these studies have been limited by the significant variability of lesion types, stage of progression, location, and rupture status of lesions (31). The variability and small sample sizes in published gene expression studies impede generalizations to the intracranial aneurysm population.
Microarray data have yielded more than 500 differentially expressed genes in intracranial aneurysm tissue (31, 110). The two most significantly associated gene ontology terms identified were in antigen processing and immune response. Additional processing of aneurysm tissue revealed significant involvement, confirmed by real-time polymerase chain reaction, in integrin signaling, chemokine signaling, complement and coagulation cascades, nitric oxide signaling, and IL-10 signaling. These studies showed a convincing correlation of major histocompatibility complex II gene overexpression in aneurysm tissue that associated antigen-presenting cells, particularly macrophages and monocytes, with intracranial aneurysm formation (31). Gene analysis of a rodent aneurysm model has shown associations in pathways involved with proteinases, reactive oxygen species, chemokines, complement, adhesion molecules, and apoptotic pathways in both the intima and media of aneurysm walls (31, 111). These data also showed differential expression of endothelial cells and VSMCs, suggesting a different role in the process of aneurysm formation (31, 112).
Gene expression patterns were more recently studied in groups of ruptured and unruptured aneurysms (31, 113), with 686 upregulated and 740 downregulated genes identified in the ruptured cohort. Upregulated pathways were numerous, most notably in response to turbulent blood flow, chemotaxis, leukocyte migration, oxidative stress, extracellular matrix degradation, and vascular remodeling. Additionally, enriched genes encoding TLR, NF-κB, hypoxia-induced factor 1A, and Ets transcription factor-binding sites were identified. These findings suggest that, although both aneurysm groups have an immunologic pedigree, ruptured and unruptured aneurysms likely have different immunologic biology.
Known genetic conditions and familial relationships are also associated with higher rates of intracranial aneurysms. Autosomal polycystic kidney disease, Ehlers–Danlos syndrome, neurofibromatosis 1, and alpha1-antitrypsin deficiency are linked with aneurysm formation (31, 40). Thus, if there are definable immunologic pathways and common identifiable genomic markers, then multiple avenues may be available for preictal intervention.
Future Directions and Treatments
Given our expanding understanding of the contribution of inflammatory factors to aneurysm formation, great efforts have been made in investigating non-interventional treatments. Much of the non-interventional therapeutic research to date has been conducted in animals, with the most promising data from studies on inhibiting the NF-κB pathway.
Multiple animal trials have sought to exploit the anti-inflammatory effect of statins. Statins can block different stages of the inflammatory reaction, decrease degeneration in the vessel, and slow intracranial aneurysm progression (3, 31, 85, 114). Unfortunately, other data indicate variable results with different doses of pravastatin (88). At lower doses (5 mg/kg/day), pravastatin reduced overall endothelial damage and inhibited aneurysm formation in rats (88). The reverse was noted at higher doses of pravastatin (25 and 50 mg/kg/day) and at lower doses of simvastatin (5 mg/kg/day), where there was enhancement of aneurysm growth, and with high-dose pravastatin, even induction of aneurysm rupture (31, 88). The adverse effects of statins were accompanied by increased apoptotic caspase-3 levels and TUNEL-positive cells. Positive but disparate results have also been found with a phosphodiesterase-4 inhibitor and several angiotensin II receptor blockers (3, 31, 81, 85, 115).
The most impressive animal data involve NF-κB inhibition in rats. A drastic decrease in inflammatory response and a 60% decrease in aneurysm incidence were found with NF-κB inhibition (31, 116). Whether the litany of animal data will have a translational impact remains to be seen. Retrospective data from the International Study of Unruptured Intracranial Aneurysms showed that patients who used aspirin three times weekly had a lower risk of aneurysm rupture versus those who did not use aspirin (117), perhaps because of the known anti-inflammatory effects of aspirin.
There are multiple, largely rat, studies of cathepsin inhibitors, MCP1 inhibitors, matrix metalloproteinase inhibitors, mast cell degranulation inhibitors, and free radical scavengers. These agents have diversely positive effects on factors, such as aneurysm incidence, size, media thickness, and internal elastic lamina score (2, 31, 97, 114, 118). The positive animal data continue to mount, prompting great hope it will translate into positive clinical therapies.
Conclusion
There is still much to learn about aneurysm biology. Experimental animal data support inflammatory pathways as a key factor in aneurysm formation, progression, and rupture, but concrete non-surgical therapeutic targets remain elusive. Continued research and understanding of the biology and immunology of aneurysms have been pivotal in broadening our current understanding and will play an important role as we continue to improve the treatment of this pathology.
Author Contributions
All authors listed have made substantial, direct, and intellectual contributions to the work and approved it for publication.
Conflict of Interest Statement
The authors declare that the research was conducted in the absence of any commercial or financial relationships that could be construed as a potential conflict of interest.
Acknowledgments
This study is supported by Barrow Neurological Institute Neuroscience Publications.
Abbreviations
IL, interleukin; NF-κB, nuclear factor-κB; SAH, subarachnoid hemorrhage; TNF, tumor necrosis factor; VSMC, vascular smooth muscle cell.
References
1. Anto RJ, Mukhopadhyay A, Shishodia S, Gairola CG, Aggarwal BB. Cigarette smoke condensate activates nuclear transcription factor-kappaB through phosphorylation and degradation of IkappaB(alpha): correlation with induction of cyclooxygenase-2. Carcinogenesis (2002) 23(9):1511–8. doi:10.1093/carcin/23.9.1511
2. Aoki T, Kataoka H, Morimoto M, Nozaki K, Hashimoto N. Macrophage-derived matrix metalloproteinase-2 and -9 promote the progression of cerebral aneurysms in rats. Stroke (2007) 38(1):162–9. doi:10.1161/01.STR.0000252129.18605.c8
3. Aoki T, Kataoka H, Ishibashi R, Nakagami H, Nozaki K, Morishita R, et al. Pitavastatin suppresses formation and progression of cerebral aneurysms through inhibition of the nuclear factor kappaB pathway. Neurosurgery (2009) 64(2):357–65; discussion 65–6. doi:10.1227/01.NEU.0000336764.92606.1D
4. Aoki T, Kataoka H, Nishimura M, Ishibashi R, Morishita R, Miyamoto S. Regression of intracranial aneurysms by simultaneous inhibition of nuclear factor-kappaB and Ets with chimeric decoy oligodeoxynucleotide treatment. Neurosurgery (2012) 70(6):1534–43; discussion 43. doi:10.1227/NEU.0b013e318246a390
5. Aoki T, Nishimura M, Kataoka H, Ishibashi R, Nozaki K, Hashimoto N. Reactive oxygen species modulate growth of cerebral aneurysms: a study using the free radical scavenger edaravone and p47phox(-/-) mice. Lab Invest (2009) 89(7):730–41. doi:10.1038/labinvest.2009.36
6. Aoki T, Nishimura M, Kataoka H, Ishibashi R, Nozaki K, Miyamoto S. Complementary inhibition of cerebral aneurysm formation by eNOS and nNOS. Lab Invest (2011) 91(4):619–26. doi:10.1038/labinvest.2010.204
7. Bruno G, Todor R, Lewis I, Chyatte D. Vascular extracellular matrix remodeling in cerebral aneurysms. J Neurosurg (1998) 89(3):431–40. doi:10.3171/jns.1998.89.3.0431
8. Chyatte D, Bruno G, Desai S, Todor DR. Inflammation and intracranial aneurysms. Neurosurgery (1999) 45(5):1137–46; discussion 46–7. doi:10.1097/00006123-199911000-00024
9. FrÖen J, Piippo A, Paetau A, Kangasniemi M, Niemela M, Hernesniemi J, et al. Growth factor receptor expression and remodeling of saccular cerebral artery aneurysm walls: implications for biological therapy preventing rupture. Neurosurgery (2006) 58(3):534–41; discussion 534–41. doi:10.1227/01.NEU.0000197332.55054.C8
10. Hoh BL, Hosaka K, Downes DP, Nowicki KW, Wilmer EN, Velat GJ, et al. Stromal cell-derived factor-1 promoted angiogenesis and inflammatory cell infiltration in aneurysm walls. J Neurosurg (2014) 120(1):73–86. doi:10.3171/2013.9.JNS122074
11. Holcomb M, Ding YH, Dai D, McDonald RJ, McDonald JS, Kallmes DF, et al. RNA-sequencing analysis of messenger RNA/MicroRNA in a rabbit aneurysm model identifies pathways and genes of interest. AJNR Am J Neuroradiol (2015) 36(9):1710–5. doi:10.3174/ajnr.A4390
12. Jayaraman T, Berenstein V, Li X, Mayer J, Silane M, Shin YS, et al. Tumor necrosis factor alpha is a key modulator of inflammation in cerebral aneurysms. Neurosurgery (2005) 57(3):558–64; discussion 558–64. doi:10.1227/01.NEU.0000170439.89041.D6
13. Jayaraman T, Paget A, Shin YS, Li X, Mayer J, Chaudhry H, et al. TNF-alpha-mediated inflammation in cerebral aneurysms: a potential link to growth and rupture. Vasc Health Risk Manag (2008) 4(4):805–17.
14. Kataoka H. Molecular mechanisms of the formation and progression of intracranial aneurysms. Neurol Med Chir (2015) 55(3):214–29. doi:10.2176/nmc.ra.2014-0337
15. Kim SC, Singh M, Huang J, Prestigiacomo CJ, Winfree CJ, Solomon RA, et al. Matrix metalloproteinase-9 in cerebral aneurysms. Neurosurgery (1997) 41(3):642–66; discussion 6–7. doi:10.1227/00006123-199709000-00027
16. Kleinloog R, Korkmaz E, Zwanenburg JJ, Kuijf HJ, Visser F, Blankena R, et al. Visualization of the aneurysm wall: a 7.0-tesla magnetic resonance imaging study. Neurosurgery (2014) 75(6):614–22; discussion 22. doi:10.1227/NEU.0000000000000559
17. Li P, Zhang Q, Wu X, Yang X, Zhang Y, Li Y, et al. Circulating microRNAs serve as novel biological markers for intracranial aneurysms. J Am Heart Assoc (2014) 3(5):e000972. doi:10.1161/JAHA.114.000972
18. Liu D, Han L, Wu X, Yang X, Zhang Q, Jiang F. Genome-wide microRNA changes in human intracranial aneurysms. BMC Neurol (2014) 14:188. doi:10.1186/s12883-014-0188-x
19. Marbacher S, FrÖen J, Marjamaa J, Anisimov A, Honkanen P, von Gunten M, et al. Intraluminal cell transplantation prevents growth and rupture in a model of rupture-prone saccular aneurysms. Stroke (2014) 45(12):3684–90. doi:10.1161/STROKEAHA.114.006600
20. Aoki T, Nishimura M, Kataoka H, Ishibashi R, Miyake T, Takagi Y, et al. Role of angiotensin II type 1 receptor in cerebral aneurysm formation in rats. Int J Mol Med (2009) 24(3):353–9.
21. Ribeiro de Sousa D, Vallecilla C, Chodzynski K, Corredor Jerez R, Malaspinas O, Eker OF, et al. Determination of a shear rate threshold for thrombus formation in intracranial aneurysms. J Neurointerv Surg (2015). 8(8):853–8. doi:10.1136/neurintsurg-2015-011737
22. Ribourtout E, Raymond J. Gene therapy and endovascular treatment of intracranial aneurysms. Stroke (2004) 35(3):786–93. doi:10.1161/01.STR.0000117577.94345.CC
23. Sawyer DM, Amenta PS, Medel R, Dumont AS. Inflammatory mediators in vascular disease: identifying promising targets for intracranial aneurysm research. Mediators Inflamm (2015) 2015:896283. doi:10.1155/2015/896283
24. Watton PN, Selimovic A, Raberger NB, Huang P, Holzapfel GA, Ventikos Y. Modelling evolution and the evolving mechanical environment of saccular cerebral aneurysms. Biomech Model Mechanobiol (2011) 10(1):109–32. doi:10.1007/s10237-010-0221-y
25. Shi C, Awad IA, Jafari N, Lin S, Du P, Hage ZA, et al. Genomics of human intracranial aneurysm wall. Stroke (2009) 40(4):1252–61. doi:10.1161/STROKEAHA.108.532036
26. Subhash N, Sriram R, Kurian GA. Sodium thiosulfate protects brain in rat model of adenine induced vascular calcification. Neurochem Int (2015) 90:193–203. doi:10.1016/j.neuint.2015.09.004
27. Szatkowska I, Szymanska O, Grabowska A. The role of the human ventromedial prefrontal cortex in memory for contextual information. Neurosci Lett (2004) 364(2):71–5. doi:10.1016/j.neulet.2004.03.084
28. Galiano G, Velasco J. Finite element approximation of a population spatial adaptation model. Math Biosci Eng (2013) 10(3):637–47. doi:10.3934/mbe.2013.10.637
29. Wang J, Yu L, Huang X, Wang Y, Zhao J. Comparative proteome analysis of saccular intracranial aneurysms with iTRAQ quantitative proteomics. J Proteomics (2016) 130:120–8. doi:10.1016/j.jprot.2015.09.014
30. Wang Y, Emeto TI, Lee J, Marshman L, Moran C, Seto SW, et al. Mouse models of intracranial aneurysm. Brain Pathol (2015) 25(3):237–47. doi:10.1111/bpa.12175
31. Chalouhi N, Ali MS, Jabbour PM, Tjoumakaris SI, Gonzalez LF, Rosenwasser RH, et al. Biology of intracranial aneurysms: role of inflammation. J Cereb Blood Flow Metab (2012) 32(9):1659–76. doi:10.1038/jcbfm.2012.84
32. Juvela S, Poussa K, Lehto H, Porras M. Natural history of unruptured intracranial aneurysms: a long-term follow-up study. Stroke (2013) 44(9):2414–21. doi:10.1161/STROKEAHA.113.001838
33. Investigators ISUIA. Unruptured intracranial aneurysms – risk of rupture and risks of surgical intervention. N Engl J Med (1998) 339(24):1725–33. doi:10.1056/NEJM199812103392401
34. Wermer MJ, van der Schaaf IC, Algra A, Rinkel GJ. Risk of rupture of unruptured intracranial aneurysms in relation to patient and aneurysm characteristics: an updated meta-analysis. Stroke (2007) 38(4):1404–10. doi:10.1161/01.STR.0000260955.51401.cd
35. Wiebers DO, Whisnant JP, Huston J III, Meissner I, Brown RD Jr, Piepgras DG, et al. Unruptured intracranial aneurysms: natural history, clinical outcome, and risks of surgical and endovascular treatment. Lancet (2003) 362(9378):103–10. doi:10.1016/S0140-6736(03)13860-3
36. Juvela S, Porras M, Poussa K. Natural history of unruptured intracranial aneurysms: probability of and risk factors for aneurysm rupture. J Neurosurg (2008) 108(5):1052–60. doi:10.3171/JNS/2008/108/5/1052
37. Brisman JL, Song JK, Newell DW. Cerebral aneurysms. N Engl J Med (2006) 355(9):928–39. doi:10.1056/NEJMra052760
38. Chalouhi N, Chitale R, Jabbour P, Tjoumakaris S, Dumont AS, Rosenwasser R, et al. The case for family screening for intracranial aneurysms. Neurosurg Focus (2011) 31(6):E8. doi:10.3171/2011.9.FOCUS11210
39. Etminan N, Macdonald RL. Computational fluid dynamics and intracranial aneurysms: higher mathematics meets complex biology. World Neurosurg (2015) 83(6):1017–9. doi:10.1016/j.wneu.2015.02.015
41. Krex D, Schackert HK, Schackert G. Genesis of cerebral aneurysms – an update. Acta Neurochir (Wien) (2001) 143(5):429–48; discussion 48–9. doi:10.1007/s007010170072
42. McDougall CG, Spetzler RF, Zabramski JM, Partovi S, Hills NK, Nakaji P, et al. The Barrow Ruptured Aneurysm Trial. J Neurosurg (2012) 116(1):135–44. doi:10.3171/2011.8.JNS101767
43. Nixon AM, Gunel M, Sumpio BE. The critical role of hemodynamics in the development of cerebral vascular disease. J Neurosurg (2010) 112(6):1240–53. doi:10.3171/2009.10.JNS09759
44. Aoki T, Nishimura M. The development and the use of experimental animal models to study the underlying mechanisms of CA formation. J Biomed Biotechnol (2011) 2011:535921. doi:10.1155/2011/535921
45. Nieuwkamp DJ, Setz LE, Algra A, Linn FH, de Rooij NK, Rinkel GJ. Changes in case fatality of aneurysmal subarachnoid haemorrhage over time, according to age, sex, and region: a meta-analysis. Lancet Neurol (2009) 8(7):635–42. doi:10.1016/S1474-4422(09)70126-7
46. Lindgaard L, Eskesen V, Gjerris F, Olsen NV. Familial aggregation of intracranial aneurysms in an Inuit patient population in Kalaallit Nunaat (Greenland). Neurosurgery (2003) 52(2):357–62; discussion 62–3. doi:10.1227/01.NEU.0000043695.77193.62
47. Nahed BV, Bydon M, Ozturk AK, Bilguvar K, Bayrakli F, Gunel M. Genetics of intracranial aneurysms. Neurosurgery (2007) 60(2):213–25; discussion 25–6. doi:10.1227/01.NEU.0000249270.18698.BB
48. Bromberg JE, Rinkel GJ, Algra A, Greebe P, van Duyn CM, Hasan D, et al. Subarachnoid haemorrhage in first and second degree relatives of patients with subarachnoid haemorrhage. BMJ (1995) 311(7000):288–9. doi:10.1136/bmj.311.7000.288
49. Ku DN. Blood flow in arteries. Annu Rev Fluid Mech (1997) 29:399–434. doi:10.1146/annurev.fluid.29.1.399
50. Ku DN, Giddens DP, Phillips DJ, Strandness DE Jr. Hemodynamics of the normal human carotid bifurcation: in vitro and in vivo studies. Ultrasound Med Biol (1985) 11(1):13–26. doi:10.1016/0301-5629(85)90003-1
51. Schulz UG, Rothwell PM. Major variation in carotid bifurcation anatomy: a possible risk factor for plaque development? Stroke (2001) 32(11):2522–9. doi:10.1161/hs1101.097391
52. Younis HF, Kaazempur-Mofrad MR, Chan RC, Isasi AG, Hinton DP, Chau AH, et al. Hemodynamics and wall mechanics in human carotid bifurcation and its consequences for atherogenesis: investigation of inter-individual variation. Biomech Model Mechanobiol (2004) 3(1):17–32. doi:10.1007/s10237-004-0046-7
53. Kapoor K, Singh B, Dewan LI. Variations in the configuration of the circle of Willis. Anat Sci Int (2008) 83(2):96–106. doi:10.1111/j.1447-073X.2007.00216.x
54. Kilic T, Sohrabifar M, Kurtkaya O, Yildirim O, Elmaci I, Gunel M, et al. Expression of structural proteins and angiogenic factors in normal arterial and unruptured and ruptured aneurysm walls. Neurosurgery (2005) 57(5):997–1007; discussion 997–1007. doi:10.1227/01.NEU.0000180812.77621.6C
55. Fukuda S, Hashimoto N, Naritomi H, Nagata I, Nozaki K, Kondo S, et al. Prevention of rat cerebral aneurysm formation by inhibition of nitric oxide synthase. Circulation (2000) 101(21):2532–8. doi:10.1161/01.CIR.101.21.2532
56. Hazama F, Hashimoto N. An animal model of cerebral aneurysms. Neuropathol Appl Neurobiol (1987) 13(2):77–90. doi:10.1111/j.1365-2990.1987.tb00173.x
57. Kim C, Cervos-Navarro J, Kikuchi H, Hashimoto N, Hazama F. Degenerative changes in the internal elastic lamina relating to the development of saccular cerebral aneurysms in rats. Acta Neurochir (1993) 121(1–2):76–81. doi:10.1007/BF01405187
58. Morimoto M, Miyamoto S, Mizoguchi A, Kume N, Kita T, Hashimoto N. Mouse model of cerebral aneurysm: experimental induction by renal hypertension and local hemodynamic changes. Stroke (2002) 33(7):1911–5. doi:10.1161/01.STR.0000021000.19637.3D
59. Nagata I, Handa H, Hashimoto N, Hazama F. Experimentally induced cerebral aneurysms in rats: Part VI. Hypertension. Surg Neurol (1980) 14(6):477–9.
60. Sadamasa N, Nozaki K, Hashimoto N. Disruption of gene for inducible nitric oxide synthase reduces progression of cerebral aneurysms. Stroke (2003) 34(12):2980–4. doi:10.1161/01.STR.0000102556.55600.3B
61. Killer-Oberpfalzer M, Aichholzer M, Weis S, Richling B, Jones R, Virmani R, et al. Histological analysis of clipped human intracranial aneurysms and parent arteries with short-term follow-up. Cardiovasc Pathol (2012) 21(4):299–306. doi:10.1016/j.carpath.2011.09.010
62. Kosierkiewicz TA, Factor SM, Dickson DW. Immunocytochemical studies of atherosclerotic lesions of cerebral berry aneurysms. J Neuropathol Exp Neurol (1994) 53(4):399–406. doi:10.1097/00005072-199407000-00012
63. Dobrin PB. Pathophysiology and pathogenesis of aortic aneurysms. Current concepts. Surg Clin North Am (1989) 69(4):687–703.
64. Nordon IM, Hinchliffe RJ, Holt PJ, Loftus IM, Thompson MM. Review of current theories for abdominal aortic aneurysm pathogenesis. Vascular (2009) 17(5):253–63. doi:10.2310/6670.2009.00046
65. Reed D, Reed C, Stemmermann G, Hayashi T. Are aortic aneurysms caused by atherosclerosis? Circulation (1992) 85(1):205–11. doi:10.1161/01.CIR.85.1.205
66. Xu XH, Shah PK, Faure E, Equils O, Thomas L, Fishbein MC, et al. Toll-like receptor-4 is expressed by macrophages in murine and human lipid-rich atherosclerotic plaques and upregulated by oxidized LDL. Circulation (2001) 104(25):3103–8. doi:10.1161/hc5001.100631
67. Ait-Oufella H, Taleb S, Mallat Z, Tedgui A. Recent advances on the role of cytokines in atherosclerosis. Arterioscler Thromb Vasc Biol (2011) 31(5):969–79. doi:10.1161/ATVBAHA.110.207415
68. Andersson J, Libby P, Hansson GK. Adaptive immunity and atherosclerosis. Clin Immunol (2010) 134(1):33–46. doi:10.1016/j.clim.2009.07.002
69. Lahoute C, Herbin O, Mallat Z, Tedgui A. Adaptive immunity in atherosclerosis: mechanisms and future therapeutic targets. Nat Rev Cardiol (2011) 8(6):348–58. doi:10.1038/nrcardio.2011.62
70. Libby P, Okamoto Y, Rocha VZ, Folco E. Inflammation in atherosclerosis: transition from theory to practice. Circ J (2010) 74(2):213–20. doi:10.1253/circj.CJ-09-0706
71. Packard RR, Libby P. Inflammation in atherosclerosis: from vascular biology to biomarker discovery and risk prediction. Clin Chem (2008) 54(1):24–38. doi:10.1373/clinchem.2007.097360
72. Packard RR, Lichtman AH, Libby P. Innate and adaptive immunity in atherosclerosis. Semin Immunopathol (2009) 31(1):5–22. doi:10.1007/s00281-009-0153-8
73. Tedgui A, Mallat Z. Cytokines in atherosclerosis: pathogenic and regulatory pathways. Physiol Rev (2006) 86(2):515–81. doi:10.1152/physrev.00024.2005
74. Abruzzo T, Shengelaia GG, Dawson RC III, Owens DS, Cawley CM, Gravanis MB. Histologic and morphologic comparison of experimental aneurysms with human intracranial aneurysms. AJNR Am J Neuroradiol (1998) 19(7):1309–14.
75. Draghia F, Draghia AC, Onicescu D. Electron microscopic study of the arterial wall in the cerebral aneurysms. Rom J Morphol Embryol (2008) 49(1):101–3.
76. Santiago-Sim T, Kim DH. Pathobiology of intracranial aneurysms. 6th ed. In: Winn HR, editor. Youmans Neurological Surgery. (Vol. 4), Philadelphia: Elsevier (2011). p. 3747–55.
77. Kataoka K, Taneda M, Asai T, Kinoshita A, Ito M, Kuroda R. Structural fragility and inflammatory response of ruptured cerebral aneurysms. A comparative study between ruptured and unruptured cerebral aneurysms. Stroke (1999) 30(7):1396–401. doi:10.1161/01.STR.30.7.1396
78. Scanarini M, Mingrino S, Giordano R, Baroni A. Histological and ultrastructural study of intracranial saccular aneurysmal wall. Acta Neurochir (1978) 43(3–4):171–82. doi:10.1007/BF01587953
79. Schlote W, Gaus C. Histologic aspects from ruptured and nonruptured aneurysms. Neurol Res (1994) 16(1):59–62.
80. Austin C, Wray S. Changes of intracellular pH in rat mesenteric vascular smooth muscle with high-K+ depolarization. J Physiol (1993) 469:1–10. doi:10.1113/jphysiol.1993.sp019800
81. Tamura T, Jamous MA, Kitazato KT, Yagi K, Tada Y, Uno M, et al. Endothelial damage due to impaired nitric oxide bioavailability triggers cerebral aneurysm formation in female rats. J Hypertens (2009) 27(6):1284–92. doi:10.1097/HJH.0b013e328329d1a7
82. Wei H, Mao Q, Liu L, Xu Y, Chen J, Jiang R, et al. Changes and function of circulating endothelial progenitor cells in patients with cerebral aneurysm. J Neurosci Res (2011) 89(11):1822–8. doi:10.1002/jnr.22696
83. Xu Y, Tian Y, Wei HJ, Chen J, Dong JF, Zacharek A, et al. Erythropoietin increases circulating endothelial progenitor cells and reduces the formation and progression of cerebral aneurysm in rats. Neuroscience (2011) 181:292–9. doi:10.1016/j.neuroscience.2011.02.051
84. Jamous MA, Nagahiro S, Kitazato KT, Satomi J, Satoh K. Role of estrogen deficiency in the formation and progression of cerebral aneurysms. Part I: experimental study of the effect of oophorectomy in rats. J Neurosurg (2005) 103(6):1046–51. doi:10.3171/jns.2005.103.6.1052
85. Kimura N, Shimizu H, Eldawoody H, Nakayama T, Saito A, Tominaga T, et al. Effect of olmesartan and pravastatin on experimental cerebral aneurysms in rats. Brain Res (2010) 1322:144–52. doi:10.1016/j.brainres.2010.01.044
86. Nagata I, Handa H, Hasimoto N, Hazama F. Experimentally induced cerebral aneurysms in rats: VII. Scanning electron microscope study. Surg Neurol (1981) 16(4):291–6. doi:10.1016/0090-3019(81)90063-X
87. Tada Y, Yagi K, Kitazato KT, Tamura T, Kinouchi T, Shimada K, et al. Reduction of endothelial tight junction proteins is related to cerebral aneurysm formation in rats. J Hypertens (2010) 28(9):1883–91. doi:10.1097/HJH
88. Tada Y, Kitazato KT, Yagi K, Shimada K, Matsushita N, Kinouchi T, et al. Statins promote the growth of experimentally induced cerebral aneurysms in estrogen-deficient rats. Stroke (2011) 42(8):2286–93. doi:10.1161/STROKEAHA.110.608034
89. Zhang D, Zhao J, Sun Y, Wang S, Tai WH, Cochrane DD, et al. Pathological observation of brain arteries and spontaneous aneurysms in hypertensive rats. Chin Med J (2003) 116(3):424–7.
90. Sakamoto N, Saito N, Han X, Ohashi T, Sato M. Effect of spatial gradient in fluid shear stress on morphological changes in endothelial cells in response to flow. Biochem Biophys Res Commun (2010) 395(2):264–9. doi:10.1016/j.bbrc.2010.04.002
91. Szymanski MP, Metaxa E, Meng H, Kolega J. Endothelial cell layer subjected to impinging flow mimicking the apex of an arterial bifurcation. Ann Biomed Eng (2008) 36(10):1681–9. doi:10.1007/s10439-008-9540-x
92. Aoki T, Nishimura M, Matsuoka T, Yamamoto K, Furuyashiki T, Kataoka H, et al. PGE(2) -EP(2) signalling in endothelium is activated by haemodynamic stress and induces cerebral aneurysm through an amplifying loop via NF-kappaB. Br J Pharmacol (2011) 163(6):1237–49. doi:10.1111/j.1476-5381.2011.01358.x
93. Owens GK. Molecular control of vascular smooth muscle cell differentiation and phenotypic plasticity. Novartis Found Symp (2007) 283:174–91; discussion 91–3, 238–41. doi:10.1002/9780470319413.ch14
94. Owens GK, Kumar MS, Wamhoff BR. Molecular regulation of vascular smooth muscle cell differentiation in development and disease. Physiol Rev (2004) 84(3):767–801. doi:10.1152/physrev.00041.2003
95. Yoshida T, Owens GK. Molecular determinants of vascular smooth muscle cell diversity. Circ Res (2005) 96(3):280–91. doi:10.1161/01.RES.0000155951.62152.2e
96. Aoki T, Kataoka H, Nishimura M, Ishibashi R, Morishita R, Miyamoto S. Ets-1 promotes the progression of cerebral aneurysm by inducing the expression of MCP-1 in vascular smooth muscle cells. Gene Ther (2010) 17(9):1117–23. doi:10.1038/gt.2010.60
97. Ishibashi R, Aoki T, Nishimura M, Hashimoto N, Miyamoto S. Contribution of mast cells to cerebral aneurysm formation. Curr Neurovasc Res (2010) 7(2):113–24. doi:10.2174/156720210791184916
98. Kolega J, Gao L, Mandelbaum M, Mocco J, Siddiqui AH, Natarajan SK, et al. Cellular and molecular responses of the basilar terminus to hemodynamics during intracranial aneurysm initiation in a rabbit model. J Vasc Res (2011) 48(5):429–42. doi:10.1159/000324840
99. Merei FT, Gallyas F. Role of the structural elements of the arterial wall in the formation and growth of intracranial saccular aneurysms. Neurol Res (1980) 2(3–4):283–303.
100. Pera J, Korostynski M, Krzyszkowski T, Czopek J, Slowik A, Dziedzic T, et al. Gene expression profiles in human ruptured and unruptured intracranial aneurysms: what is the role of inflammation? Stroke (2010) 41(2):224–31. doi:10.1161/STROKEAHA.109.562009
101. Sakaki T, Kohmura E, Kishiguchi T, Yuguchi T, Yamashita T, Hayakawa T. Loss and apoptosis of smooth muscle cells in intracranial aneurysms. Studies with in situ DNA end labeling and antibody against single-stranded DNA. Acta Neurochir (Wien) (1997) 139(5):469–74; discussion 74–5. doi:10.1007/BF01808885
102. Sibon I, Mercier N, Darret D, Lacolley P, Lamaziere JM. Association between semicarbazide-sensitive amine oxidase, a regulator of the glucose transporter, and elastic lamellae thinning during experimental cerebral aneurysm development: laboratory investigation. J Neurosurg (2008) 108(3):558–66. doi:10.3171/JNS/2008/108/3/0558
103. Aoki T, Kataoka H, Moriwaki T, Nozaki K, Hashimoto N. Role of TIMP-1 and TIMP-2 in the progression of cerebral aneurysms. Stroke (2007) 38(8):2337–45. doi:10.1161/STROKEAHA.107.481838
104. Nakajima N, Nagahiro S, Sano T, Satomi J, Satoh K. Phenotypic modulation of smooth muscle cells in human cerebral aneurysmal walls. Acta Neuropathol (2000) 100(5):475–80. doi:10.1007/s004010000220
105. Tada S, Tarbell JM. Interstitial flow through the internal elastic lamina affects shear stress on arterial smooth muscle cells. Am J Physiol Heart Circ Physiol (2000) 278(5):H1589–97.
106. Aoki T, Kataoka H, Ishibashi R, Nozaki K, Morishita R, Hashimoto N. Reduced collagen biosynthesis is the hallmark of cerebral aneurysm: contribution of interleukin-1beta and nuclear factor-kappaB. Arterioscler Thromb Vasc Biol (2009) 29(7):1080–6. doi:10.1161/ATVBAHA.108.180760
107. Jamous MA, Nagahiro S, Kitazato KT, Tamura T, Aziz HA, Shono M, et al. Endothelial injury and inflammatory response induced by hemodynamic changes preceding intracranial aneurysm formation: experimental study in rats. J Neurosurg (2007) 107(2):405–11. doi:10.3171/JNS-07/08/0405
108. Kadirvel R, Ding YH, Dai D, Zakaria H, Robertson AM, Danielson MA, et al. The influence of hemodynamic forces on biomarkers in the walls of elastase-induced aneurysms in rabbits. Neuroradiology (2007) 49(12):1041–53. doi:10.1007/s00234-007-0295-0
109. Wang Y, Zheng XR, Riddick N, Bryden M, Baur W, Zhang X, et al. ROCK isoform regulation of myosin phosphatase and contractility in vascular smooth muscle cells. Circ Res (2009) 104(4):531–40. doi:10.1161/CIRCRESAHA.108.188524
110. Krischek B, Kasuya H, Tajima A, Akagawa H, Sasaki T, Yoneyama T, et al. Network-based gene expression analysis of intracranial aneurysm tissue reveals role of antigen presenting cells. Neuroscience (2008) 154(4):1398–407. doi:10.1016/j.neuroscience.2008.04.049
111. Aoki T, Kataoka H, Ishibashi R, Nozaki K, Hashimoto N. Gene expression profile of the intima and media of experimentally induced cerebral aneurysms in rats by laser-microdissection and microarray techniques. Int J Mol Med (2008) 22(5):595–603.
112. Aoki T, Kataoka H, Ishibashi R, Nozaki K, Hashimoto N. Simvastatin suppresses the progression of experimentally induced cerebral aneurysms in rats. Stroke (2008) 39(4):1276–85. doi:10.1161/STROKEAHA.107.503086
113. Kurki MI, Gaal EI, Kettunen J, Lappalainen T, Menelaou A, Anttila V, et al. High-risk population isolate reveals low frequency variants predisposing to intracranial aneurysms. PLoS Genet (2014) 10(1):e1004134. doi:10.1371/journal.pgen.1004134
114. Aoki T, Kataoka H, Ishibashi R, Nozaki K, Hashimoto N. Cathepsin B, K, and S are expressed in cerebral aneurysms and promote the progression of cerebral aneurysms. Stroke (2008) 39(9):2603–10. doi:10.1161/STROKEAHA.107.513648
115. Yagi K, Tada Y, Kitazato KT, Tamura T, Satomi J, Nagahiro S. Ibudilast inhibits cerebral aneurysms by down-regulating inflammation-related molecules in the vascular wall of rats. Neurosurgery (2010) 66(3):551–9; discussion 9. doi:10.1227/01.NEU.0000365771.89576.77
116. Aoki T, Kataoka H, Shimamura M, Nakagami H, Wakayama K, Moriwaki T, et al. NF-kappaB is a key mediator of cerebral aneurysm formation. Circulation (2007) 116(24):2830–40. doi:10.1161/CIRCULATIONAHA.107.728303
117. Hasan DM, Mahaney KB, Brown RD Jr, Meissner I, Piepgras DG, Huston J, et al. Aspirin as a promising agent for decreasing incidence of cerebral aneurysm rupture. Stroke (2011) 42(11):3156–62. doi:10.1161/STROKEAHA.111.619411
Keywords: biology, inflammation, intracranial aneurysm
Citation: Fennell VS, Kalani MYS, Atwal G, Martirosyan NL and Spetzler RF (2016) Biology of Saccular Cerebral Aneurysms: A Review of Current Understanding and Future Directions. Front. Surg. 3:43. doi: 10.3389/fsurg.2016.00043
Received: 25 March 2016; Accepted: 06 July 2016;
Published: 25 July 2016
Edited by:
Eberval Figueiredo, Hospital das Clinicas University of Sao Paulo, BrazilReviewed by:
Jorge Marcelo Mura, Institute of Neurosurgery Asenjo, ChileYasunori Fujimoto, Osaka University, Japan
Sabrina Degaspari, São Paulo University, Brazil
Copyright: © 2016 Fennell, Kalani, Atwal, Martirosyan and Spetzler. This is an open-access article distributed under the terms of the Creative Commons Attribution License (CC BY). The use, distribution or reproduction in other forums is permitted, provided the original author(s) or licensor are credited and that the original publication in this journal is cited, in accordance with accepted academic practice. No use, distribution or reproduction is permitted which does not comply with these terms.
*Correspondence: Robert F. Spetzler, neuropub@dignityhealth.org